How To Build A Nuclear Weapon
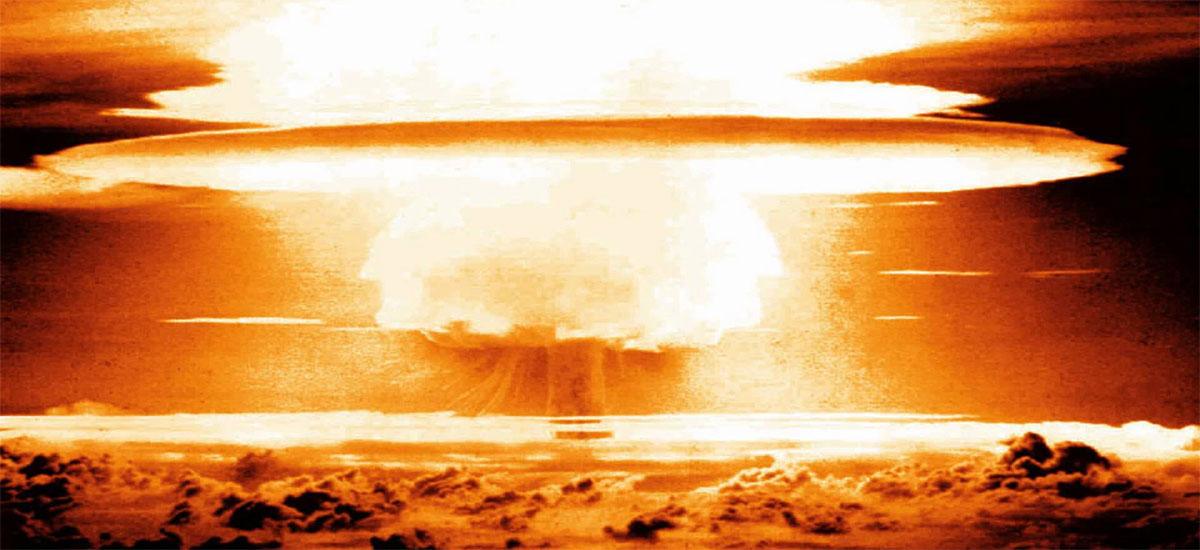
Who hasn't dreamed of constructing a massive atomic bomb in their own underground lair somewhere in the mountains? Well, first you need to understand the theory and mechanics of how atoms destroy entire cities. For more, a great read is Weapons of Tomorrow by Brian Beckett (Plenum Press, 1983).
Atoms 101: What Are They?
Atoms are the basic building blocks of life, and the different ones are known as elements. Each is composed of protons (positively-charged particles), electrons (negatively-charged particles) and neutrons (particles which aren't positive or negative).
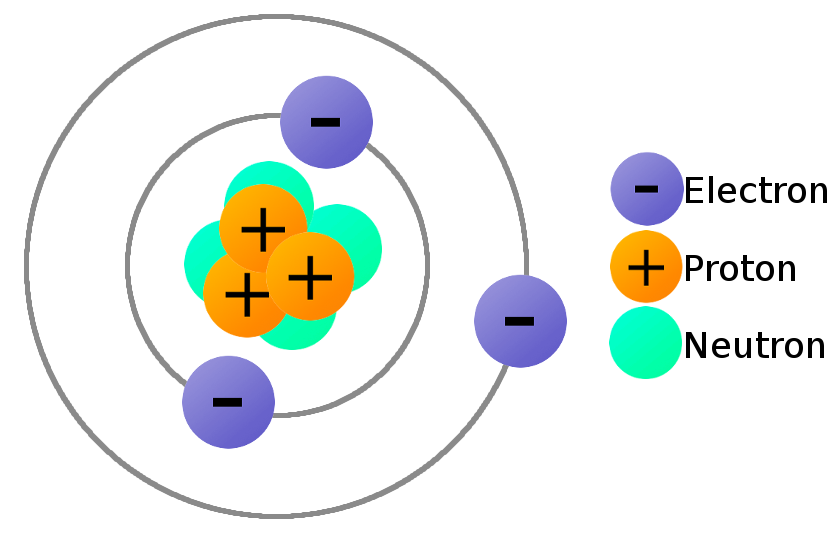
Neutrons and protons (nucleons) are roughly similar in size and live in the atom's nucleus. Electrons are 1,836x smaller and orbit the nucleus.
Elements are given a symbol abbreviation and organised in a chart named the Periodic Table. They are numbered according to the amount of protons they have. It starts with Hydrogen (H), which has an atomic number of 1 because each atom of hydrogen has 1 proton.
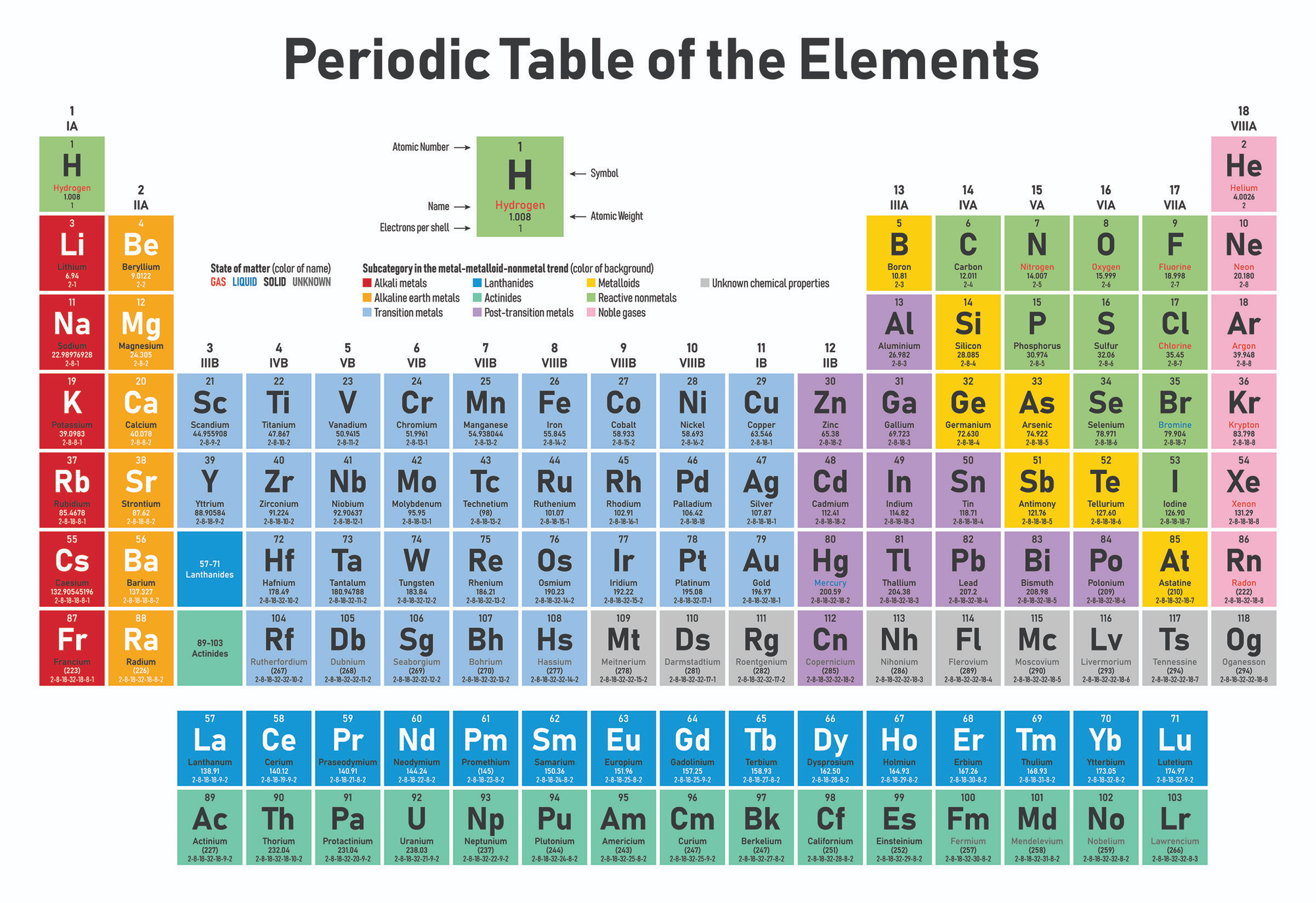
Carbon has an atomic number of 6, because it has 6 protons (6 positively charged particles). It has 6 neutrons (no charge), and 6 electrons (negative charge).
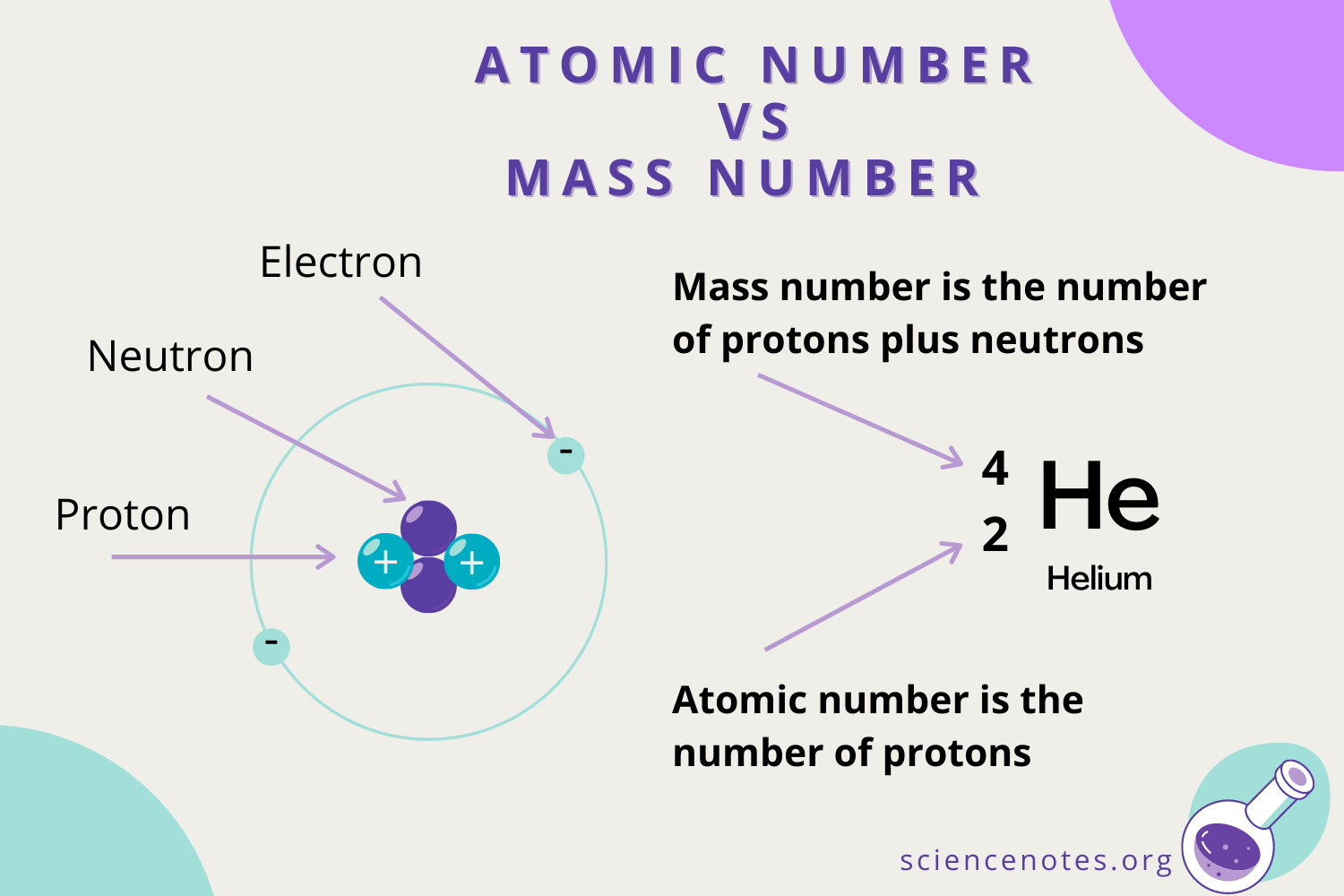
At the other end, Moscovium has an atomic number of 115, meaning it has 115 protons (positive charge), 115 electrons (negative charge), and the number of neutrons in an atom can be determined by the difference between the atomic mass and the number of protons. The difference between the mass number of the Moscovium atom (290) and the number of protons (115) is 175 (i.e. 290 - 115). Therefore, a Moscovium atom has 175 neutrons.
Which Atom Can We Split?
The scientists who discovered the atom could be split, Otto Hahn and Fritz Strassmann, made the breakthrough in 1938, and it was later explained theoretically by Lise Meitner and Otto Frisch.
Nuclear weapons start with fission, which means to split an atom. Atoms do not split easily, but when they do, they release an enormous amount of energy.
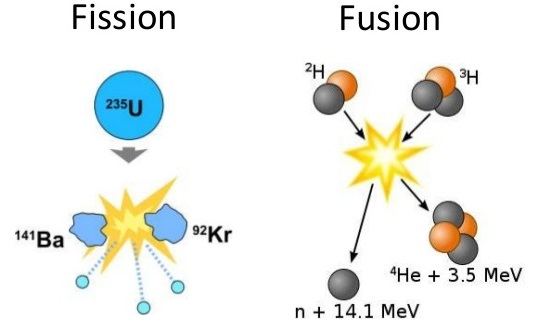
Obviously it must be naturally-occurring so we can mine it cost-effectively.
The first thing we need is a elements with a high atomic number, which releases a high yield of energy. A larger nuclei, makes an atom more susceptible to fission when bombarded with neutrons, because it provides a bigger "target" for incoming neutrons, making the neutron capture process more likely.
As nuclei get larger (i.e., as you move up the periodic table toward higher atomic numbers), the balance between the attractive strong nuclear force and the repulsive electrostatic force (from the protons) becomes more precarious. A high atomic number means more protons in the nucleus, leading to increased repulsion. This makes larger nuclei like those of uranium less stable and more susceptible to fission.
Secondly, it must be fissile, to sustain a chain reaction when bombarded with thermal (slow-moving) neutrons; meaning it releases, on average, more than two additional neutrons. If these neutrons go on to cause further fissions, a chain reaction can be sustained.
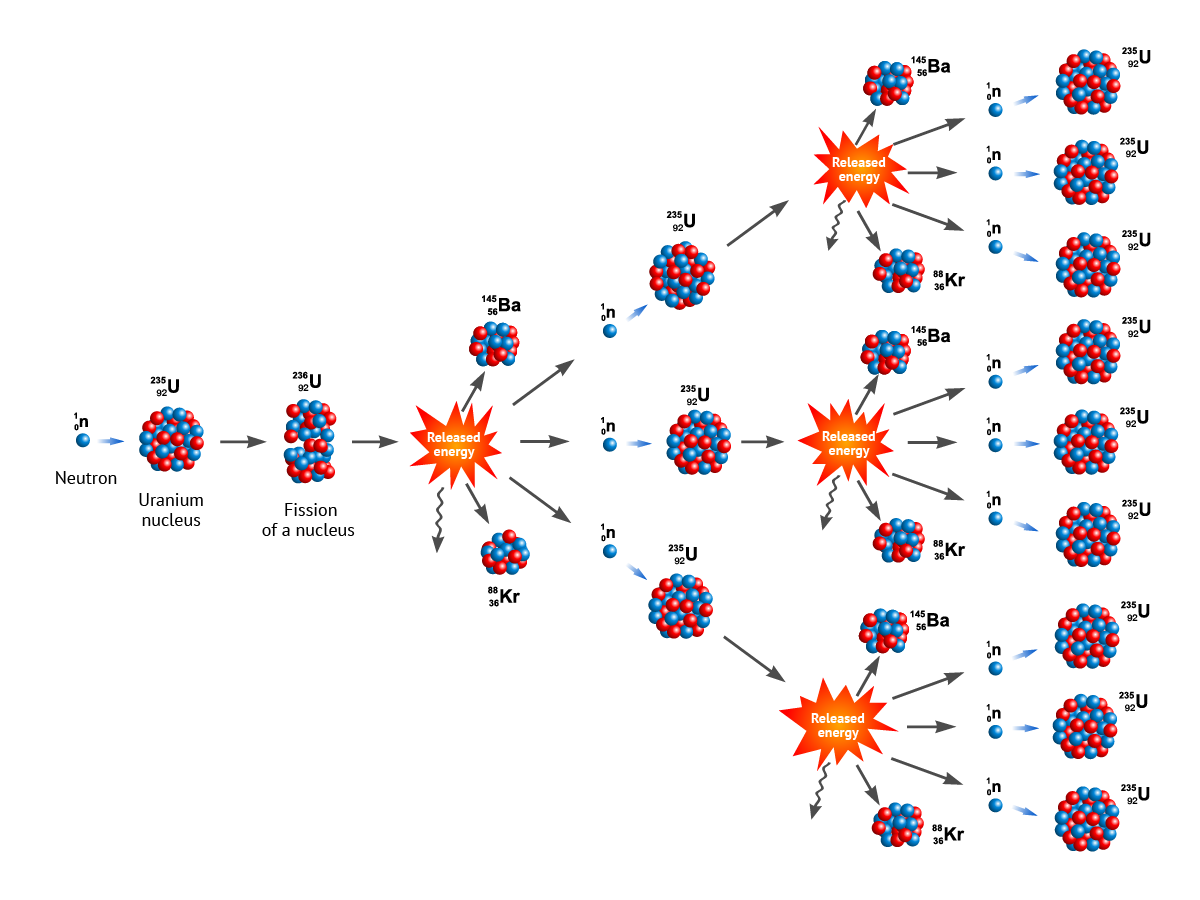
The fission of heavy nuclei typically results in medium-sized daughter nuclei. The binding energy per nucleon (a measure of nuclear stability) is highest for medium-sized nuclei (around the size of iron). When a large nucleus splits into medium-sized nuclei, the resulting nuclei are more tightly bound than the original large nucleus. This difference in binding energy is released as kinetic energy, which is the energy harnessed in nuclear reactors.
Thirdly, it must be fertile and degrade to a material which is also splittable (fissile) as a form of "breeding" more fuel.
The key elements that can undergo fission, by atomic number:
Thorium (90):
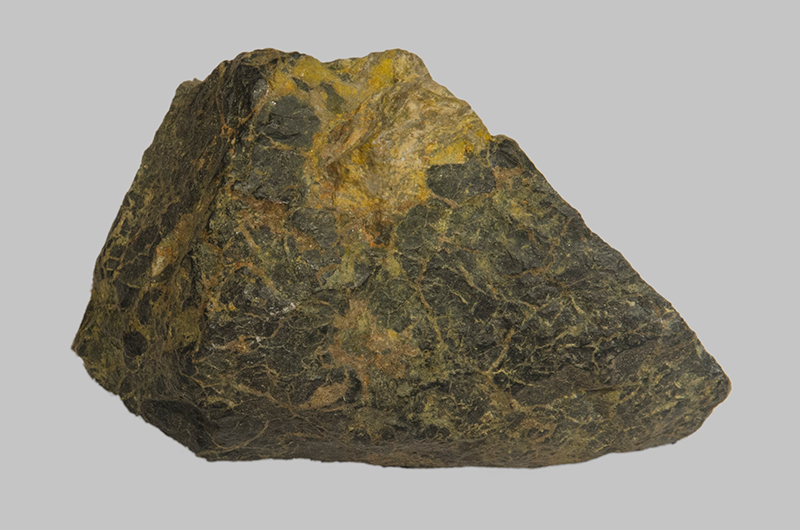
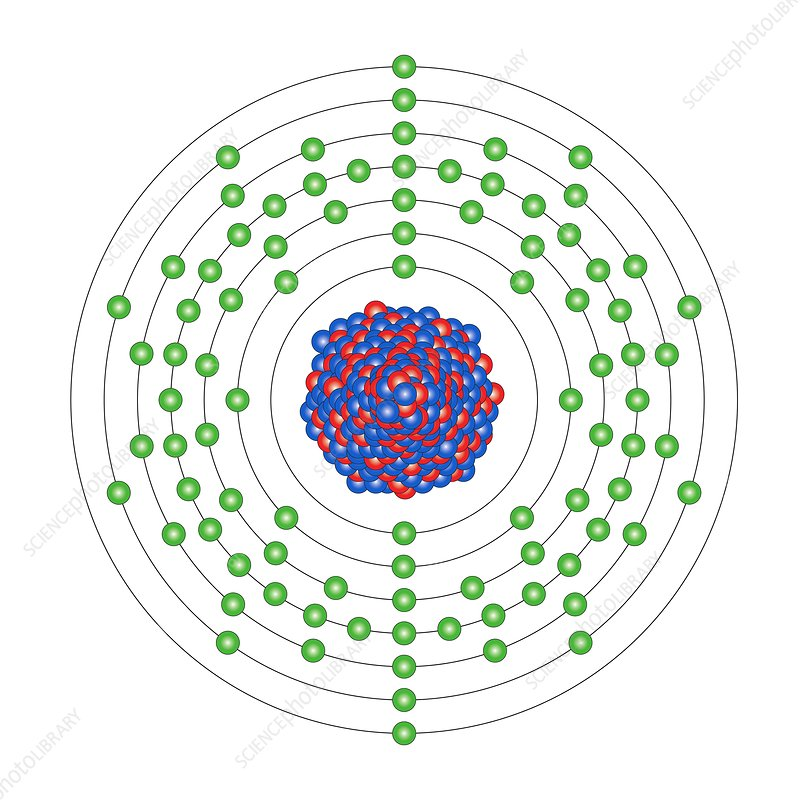
- Th-232: Not directly fissile, but when it captures a neutron, it is converted into U-233, which is fissile.
Uranium (92):
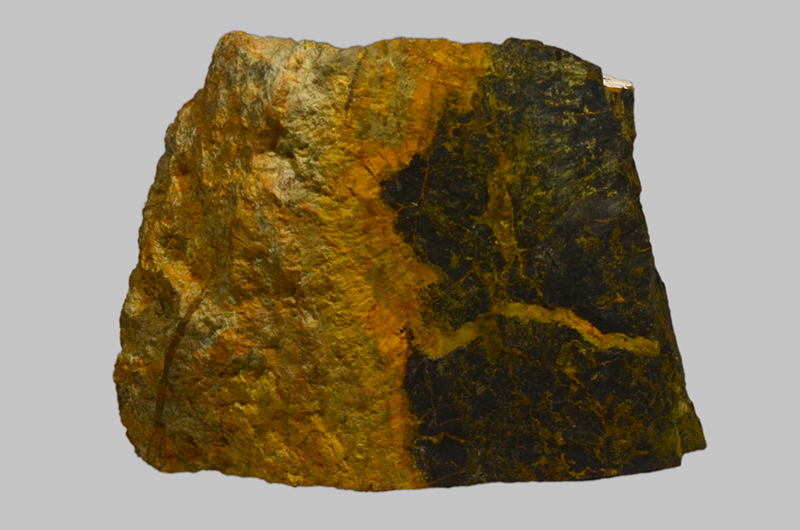
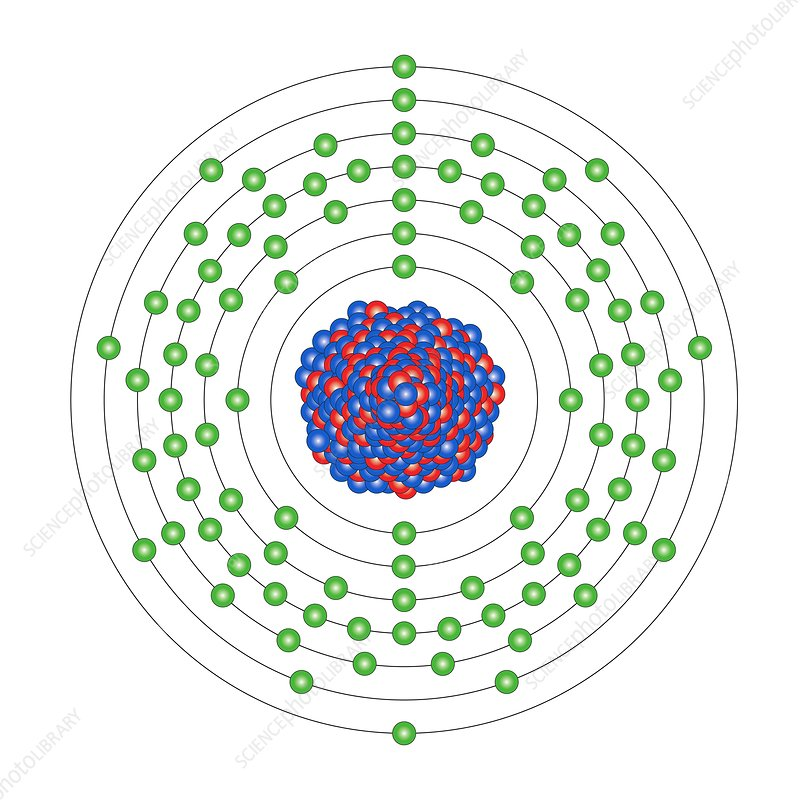
- U-235: Naturally occurring and fissile. It's the primary fuel in many nuclear reactors and can sustain a chain reaction with thermal (slow) neutrons.
- U-238: While not fissile with thermal neutrons, it can undergo fast fission with high-energy (fast) neutrons. Moreover, it can be converted to Pu-239, which is fissile, when it captures a neutron.
Plutonium (94):
This element has the highest atomic number found in nature, and is produced in nuclear reactors.
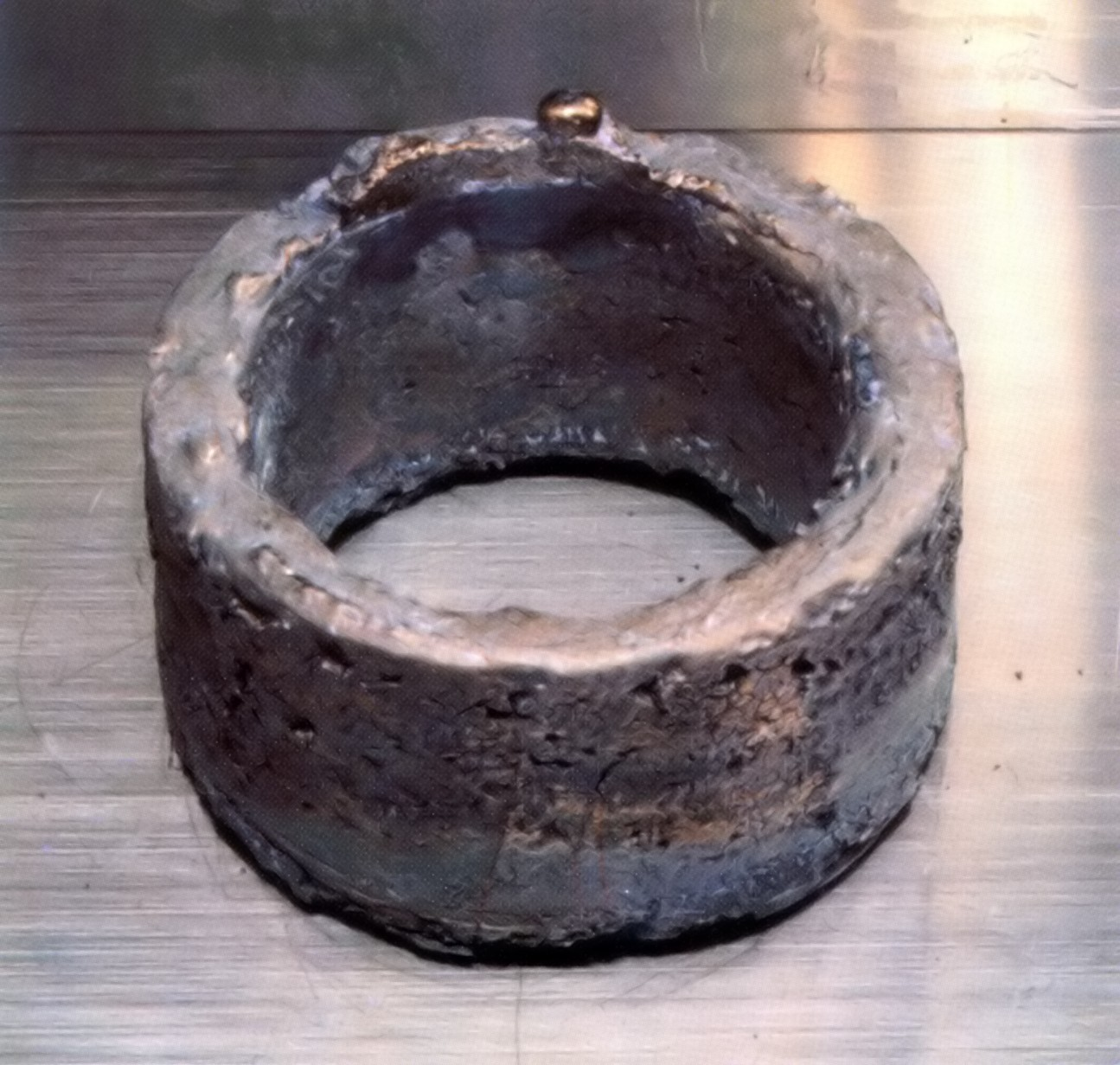
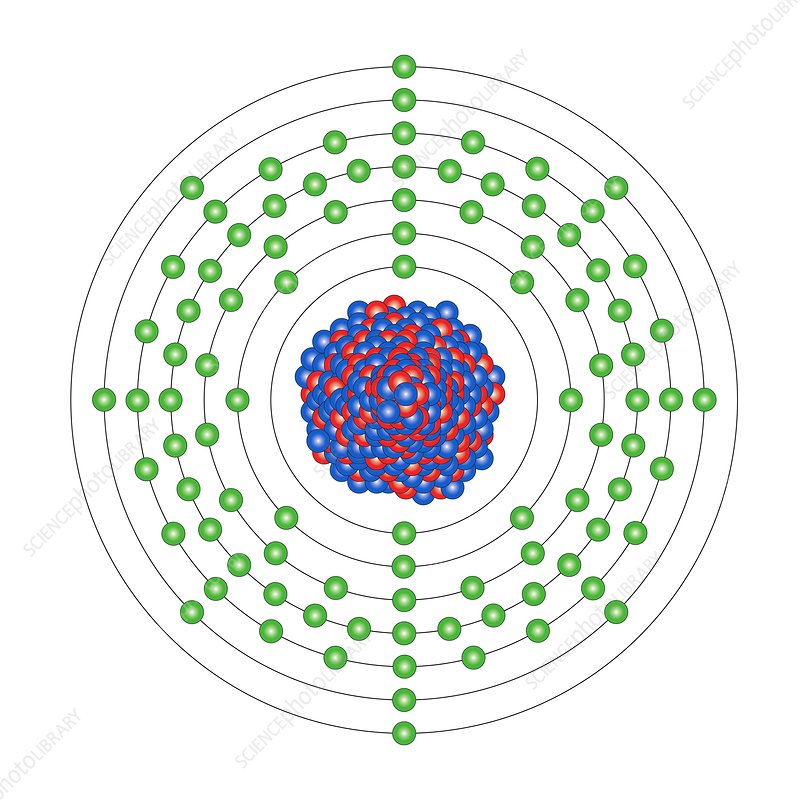
- Pu-239: Fissile and is used both as a nuclear reactor fuel (especially in fast breeder reactors) and in the production of nuclear weapons.
- Pu-240: Can undergo fast fission but is not as easily fissile as Pu-239.
Neptunium (93):
- Np-239: Can undergo fission and can also decay into Pu-239.
- Americium (95), Curium (96), and other Transuranic Elements: Some isotopes of these heavy, man-made elements can undergo fission, especially when bombarded with fast neutrons.
- Fission Fragments: The products of fission (known as fission fragments) can sometimes be induced to fission themselves, especially if they are neutron-rich and hit with additional neutrons.
For a nuclear reactor or a bomb, the ability to sustain a chain reaction is crucial. This requires the material to not only undergo fission but also to emit, on average, more than one neutron per fission event that can cause subsequent fissions. U-235, Pu-239, and U-233 are especially noteworthy because they can sustain a chain reaction with thermal neutrons, making reactor design more feasible.
How Do We Split It?
In essence, we need to smash a bunch of material into more material. Really, really fast. We slam a load of atoms into other atoms, Wile E Coyote style, and hope for the best.
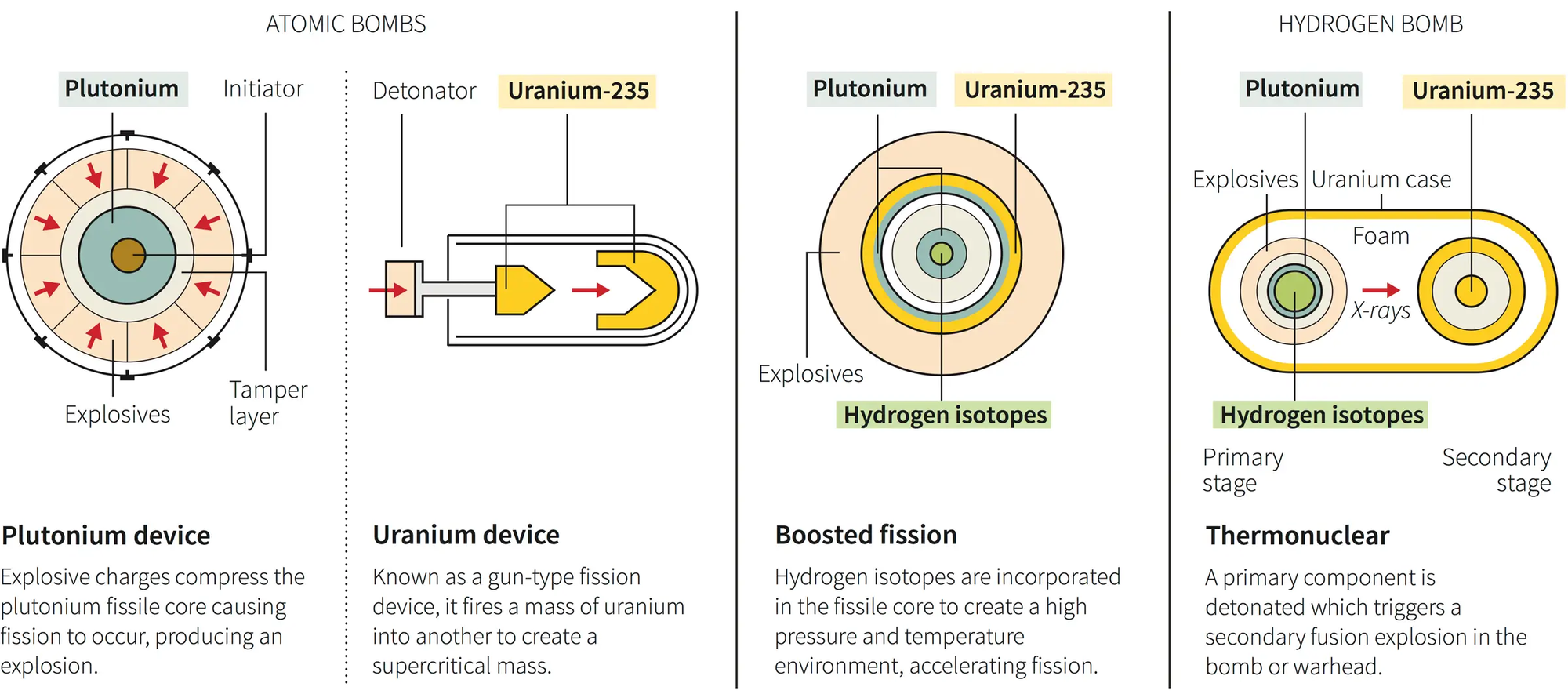
There are two ways, both of which require an initial explosive charge: shooting like a bullet from a gun, and imploding down into a ball.
Both methods aim to bring a sub-critical mass of fissile material to a supercritical state rapidly enough to initiate a fission chain reaction before the assembly blows itself apart. This chain reaction releases an enormous amount of energy in the form of a nuclear explosion.
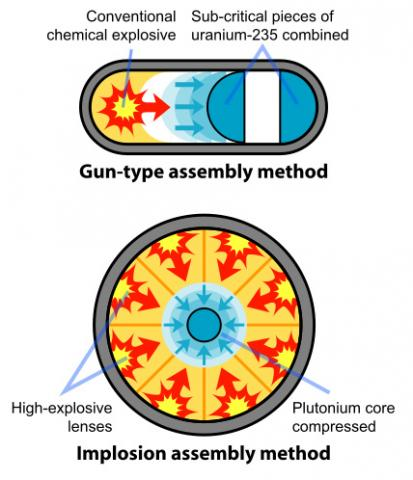
Hiroshima "Little Boy": the Gun Method
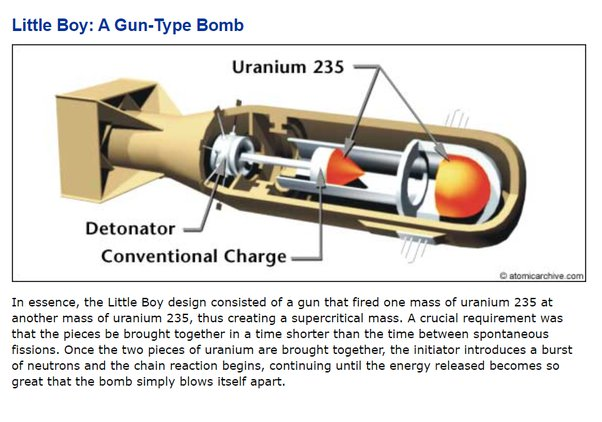
In this method, two sub-critical pieces of fissile material are brought together rapidly to form a supercritical mass, initiating a chain reaction. This method is less efficient, requires more fissile material, and is generally easier to detect and counter. Additionally, it is typically limited to uranium-235 as the fissile material.
One piece of sub-critical fissile material is shot, like a bullet, down a barrel into another piece of fissile material using conventional explosives.
The rapid assembly into a supercritical mass allows for a sustained chain reaction before the assembly can disassemble itself, resulting in a nuclear explosion.
This 15kt bomb used 64kg (51kg U235) of highly-enriched uranium (HEU) and had a yield of 1.7%.
Nagasaki "Fat Man": the Implosion Method
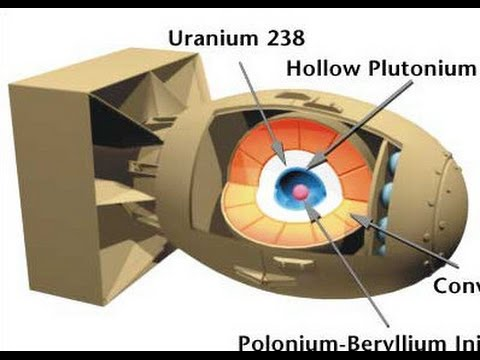
A sub-critical sphere (or shell) of fissile material is surrounded by high explosives. This method is more efficient, requires less fissile material, and can be used with plutonium-239 as well as uranium-235.
The explosives are detonated symmetrically, compressing the fissile material into a supercritical mass, which then initiates the chain reaction. All the explosives must fire inwardly at the same exact microsecond.
When detonated, these explosives compress the plutonium into a supercritical state. The compression happens so fast that it allows for a chain reaction to occur before the fissile material can blow itself apart.
This 20kt bomb used 6.2kg of Plutonium and had a yield of 18%.
The released energy is distributed as:
- 50% blast
- 35% thermal radiation (heat)
- 10% residual radiation (fallout)
- 5 % prompt radiation (gamma rays, neutrons, X-rays)
How Do We Get A Chain Reaction?
During the Manhattan Project, scientists like Enrico Fermi worked on calculating the amount of fissile material that would be needed to sustain a chain reaction. Experiments, like the "Chicago Pile-1", were essential in understanding the conditions under which a controlled chain reaction could occur.
The transition from a controlled reaction (like in a nuclear reactor) to an uncontrolled, rapid reaction (as in a nuclear weapon) required further calculations and experiments, many of which were classified and remain so. The critical mass varies depending on the material (uranium-235 or plutonium-239), its purity, its shape, and other factors like the presence of a "tamper" to reflect neutrons back into the fissile core.
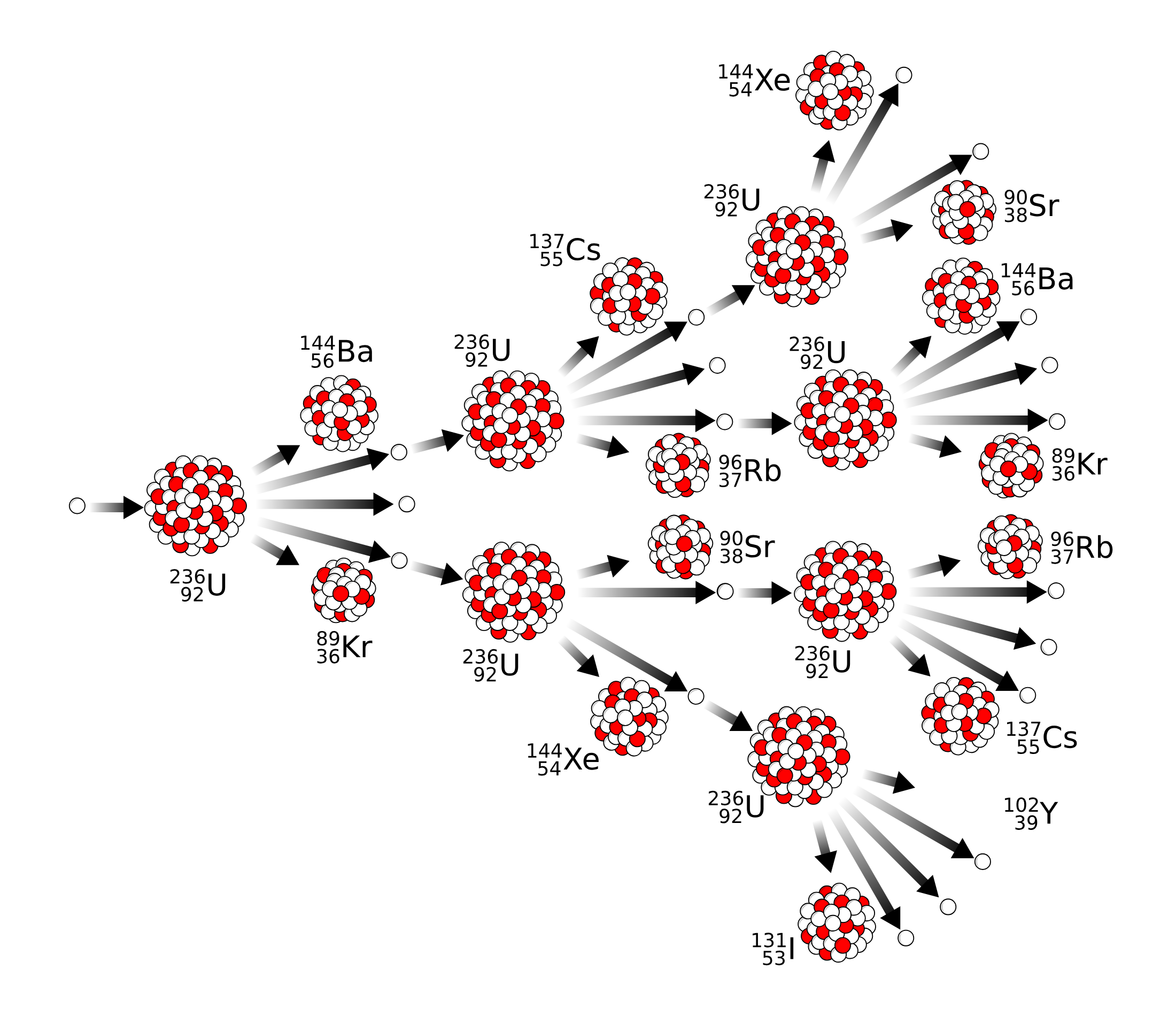
- Initiation: A high-energy neutron collides with a heavy fissile nucleus, such as uranium-235 or plutonium-239. This makes the nucleus unstable.
- Fission: The unstable nucleus splits into two or more smaller nuclei, releasing a large amount of energy in the form of heat and radiation.
- Neutron Emission: The fission process also emits additional high-energy neutrons—usually two or three but possibly more.
- Propagation: These newly emitted neutrons can then collide with other fissile nuclei, causing them to fission as well and releasing even more neutrons.
- Chain Reaction: This process can continue, with each fission event producing more neutrons that cause more fissions. The result is an exponential increase in the number of fission events and, consequently, an enormous release of energy.
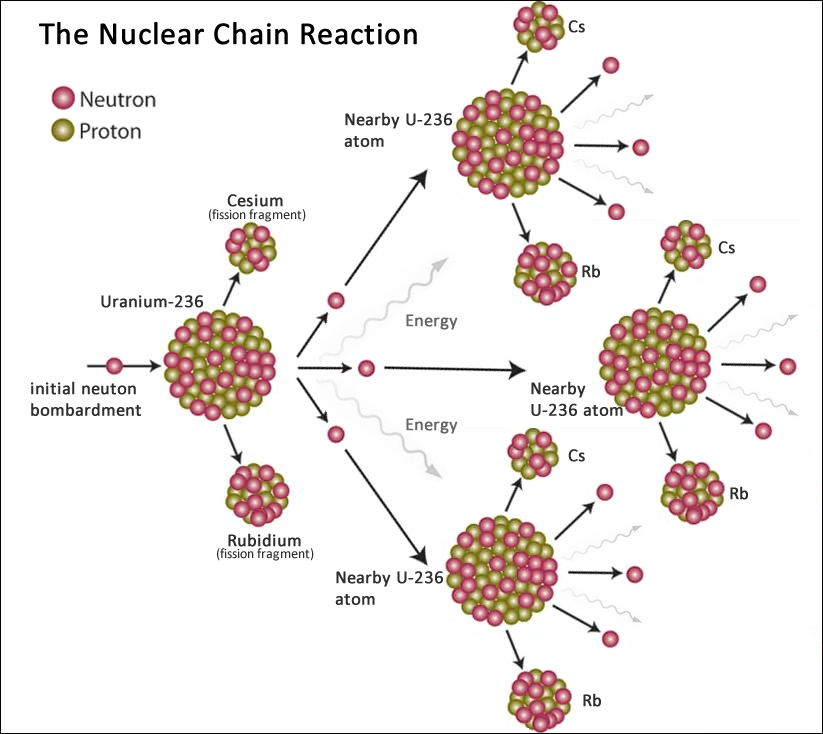
In a controlled setting like a nuclear reactor, various methods (e.g., control rods, coolant) are used to absorb some of the neutrons and regulate the chain reaction. In an uncontrolled setting like a nuclear bomb, the aim is to allow the chain reaction to occur as rapidly and completely as possible before the assembly blows itself apart.
For a chain reaction to be self-sustaining, the system needs to reach "criticality," where each fission event, on average, produces at least one additional fission event. Sub-critical systems die out, while supercritical systems grow exponentially.
The first 25kt atomic bomb ("Trinity", or the "Gadget") was detonated by the US Army 5:29 a.m. MWT (11:29:21 GMT) on July 16, 1945, 35 miles (56 km) southeast of Socorro, New Mexico, on what was the Alamogordo Bombing and Gunnery Range (renamed the White Sands Proving Ground just before the test). GPS coords: https://goo.gl/maps/JeTxQBwF5etp8pjq7
How Do We Enhance the Reaction?
To concentrate the reaction and make it more explosive, you need what is known as a tamper, which serves as a shell surrounding the fissile core.
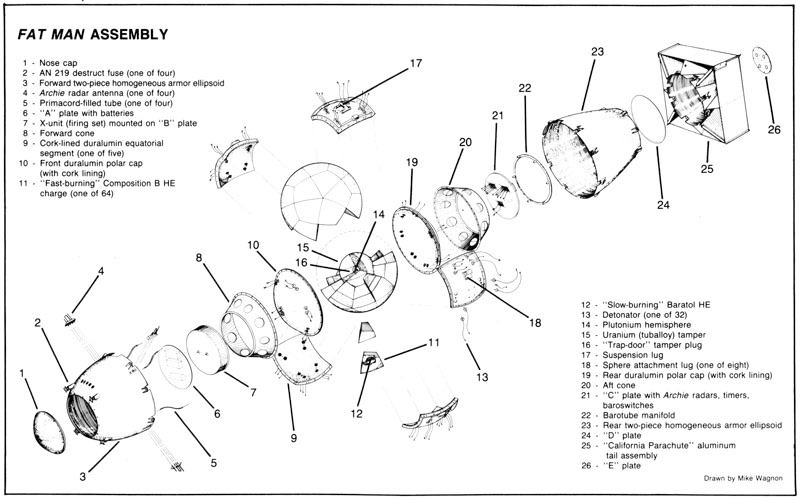
The tamper - "compressor" or "reflector" - reflects some of the neutrons emitted during the fission process back into the fissile material, increasing the likelihood of additional fission reactions and thereby enhancing the weapon's yield.
In implosion-type devices like the Nagasaki bomb, the tamper also helps to maintain the compression of the fissile material for a longer period, enabling a more sustained and efficient chain reaction.
How Do We Produce the Fuel?
Uranium and Plutonium come as a mix of isotopes, and we need to separate the rare, specific fissile isotopes we need: U-235 and Pu-239. Natural Uranium is 0.72% of its natural ore. Plutonium is produced in nuclear reactors (as spent fuel) by adding neutrons to Uranium-238.
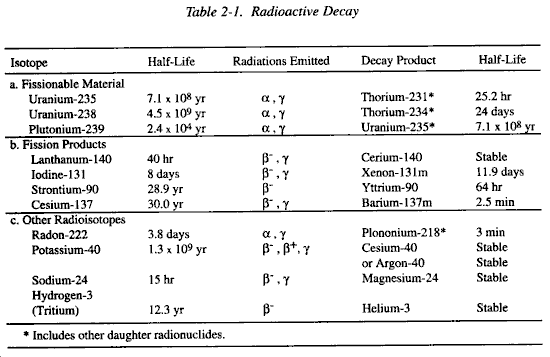
Nuclear fuels come in different grades. Weapons can be made without weapons-grade purity, but it's unusual.
- Depleted (< 1% purity)
- Low-enriched (< 5% purity)
- Fuel grade (7-18% purity)
- Reactor or highly-enriched grade (18%+ purity)
- Weapons-grade (varying, typically 90%+ purity)
- Supergrade (90%+)
Highly-enriched Uranium (HEU) is at least 20% U-235. Bombs typically require 90%+ purity, either Uranium ($52/lb) or Plutonium ($2.6M/lb).
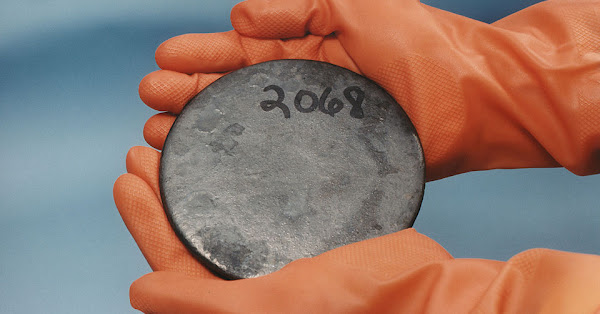
Plutonium-239 is produced by recycling spent fuel from nuclear reactors, making it extremely expensive but slightly easier. Uranium-235 is produced by refining and purifying Uranium ore in a process known as enrichment. Both are extremely labour-intensive and technically difficult.
The key issue is the knowledge and personnel to do it. 90% of the effort of the Manhattan Project was making the fuel.
Setting up an enrichment facility is a complex and resource-intensive task that requires advanced technology and expertise. However, the spread of knowledge and technology, coupled with the potential for illicit trade, makes it increasingly feasible for rogue states to pursue such activities. Monitoring and non-proliferation efforts by international bodies like the International Atomic Energy Agency (IAEA) are essential for tracking and inhibiting these activities.
Gaseous Diffusion
Uuranium hexafluoride gas is forced through a series of porous membranes. U-235 molecules pass through slightly more easily than U-238, gradually increasing the concentration of U-235.
Gaseous diffusion is one of the older methods for uranium enrichment, although it has largely been superseded by more efficient techniques like gas centrifugation. The process focuses on the slight mass difference between uranium-235 (U-235) and uranium-238 (U-238) to separate these isotopes.
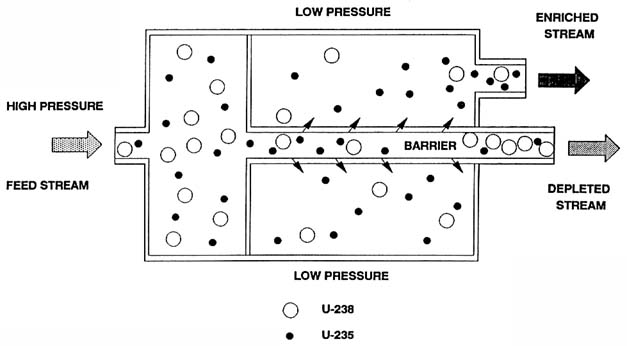
- Conversion to Gas: Initially, natural uranium ore is processed to produce uranium hexafluoride (UF6). Both U-235 and U-238 are converted into this gaseous compound.
- Preparation of Membrane: A porous barrier or membrane is prepared, which has holes that are just large enough to allow UF6 gas molecules to pass through.
- Pressurization: The UF6 gas is pressurized and forced against the membrane.
- Diffusion: Due to their slight difference in mass, the lighter U-235 hexafluoride molecules diffuse through the membrane slightly more readily than the heavier U-238 hexafluoride molecules. The gas that makes it through the barrier is slightly enriched in U-235 compared to the gas that didn't pass through.
- Cascade: Because each pass through a single barrier provides only a small amount of enrichment, a cascade of barriers is used. Gas flows from one barrier to the next, becoming incrementally more enriched at each stage.
- Recirculation: The process is highly repetitive; the gas must pass through many stages of diffusion (often thousands) to reach the desired level of enrichment.
- Withdrawal and Conversion: Once the gas reaches the desired enrichment level, it is withdrawn from the cascade. The enriched UF6 can then be converted back into a more stable form of uranium for use in nuclear reactors or, at very high levels of enrichment, in nuclear weapons.
Gaseous diffusion is highly energy-intensive due to the need for pressurizing the gas and maintaining the cascade. The process also takes a long time, making it less efficient compared to newer methods like gas centrifugation.
Despite its drawbacks, gaseous diffusion was one of the first methods developed for isotope separation and was used extensively during the Manhattan Project and the early years of nuclear energy and weapons development.
Gas Centrifuge
A more modern and efficient method involves spinning uranium hexafluoride gas in centrifuges. The lighter U-235 isotopes concentrate near the center of the rotation, while the heavier U-238 isotopes are pushed toward the outer walls.
The gas centrifuge method is one of the most widely used and efficient techniques for enriching uranium. This method also relies on the small mass difference between the isotopes uranium-235 (U-235) and uranium-238 (U-238).
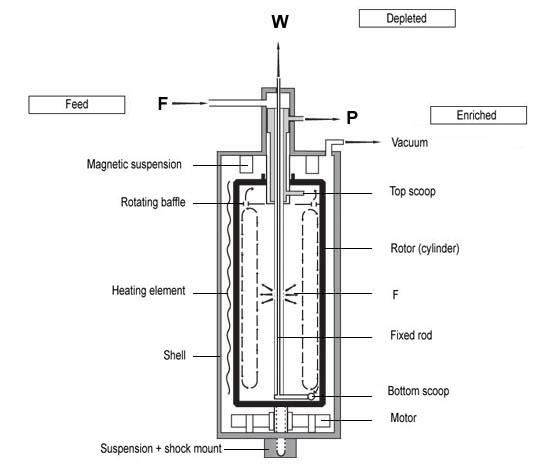
- Conversion to Gas: First, uranium ore is processed to produce uranium hexafluoride (UF6), a compound that can exist in gaseous form under suitable conditions.
- Centrifuge Construction: The centrifuges themselves are cylindrical devices made of strong, lightweight materials like carbon fiber composites or specialized steel alloys to withstand high rotational speeds.
- Spinning: UF6 gas is fed into a rapidly spinning centrifuge. Due to centrifugal force, the heavier U-238 isotope tends to move toward the outer wall of the cylinder, while the lighter U-235 isotope stays closer to the axis of rotation.
- Separation and Collection: A scoop or similar device collects enriched UF6 gas from the area closer to the center (enriched in U-235) and depleted UF6 gas from the area closer to the wall (depleted in U-235).
- Cascade: Similar to the gaseous diffusion method, a single centrifuge provides only a slight increase in enrichment. For significant enrichment, centrifuges are connected in a cascade. The slightly enriched UF6 from one centrifuge feeds into the next, and the process is repeated through many stages.
- Further Enrichment or Use: The enriched gas can be further processed or converted back into a more solid form of uranium for use in reactors. If the enrichment level is significantly high, it could theoretically be used for weapons, which is why the technology is highly controlled.
It has advantages over diffusion.
- Energy-Efficiency: Gas centrifuges use significantly less energy compared to gaseous diffusion, making them more cost-effective.
- Speed and Efficiency: The centrifuge process is faster and more efficient in terms of the amount of enriched uranium produced over time.
- Scalability: The modular nature of the centrifuge method allows for easier scaling, as more centrifuges can be added to existing facilities to increase capacity.
Because of its efficiency and lower energy requirements, the gas centrifuge method has largely replaced gaseous diffusion for uranium enrichment. However, the technology and materials for building centrifuges are tightly controlled due to the potential for misuse in the production of highly enriched uranium for nuclear weapons. Monitoring and oversight by international bodies like the International Atomic Energy Agency (IAEA) are critical for ensuring that centrifuge technology is used only for peaceful purposes.
Laser Isotope Separation
This is a less commonly used method where lasers are employed to selectively ionize U-235, making it easier to separate from U-238.
The process uses lasers to selectively ionize or excite specific isotopes of uranium, making it easier to separate them from each other. There are several variants of LIS, but two of the most known methods are Atomic Vapor Laser Isotope Separation (AVLIS) and Molecular Laser Isotope Separation (MLIS).
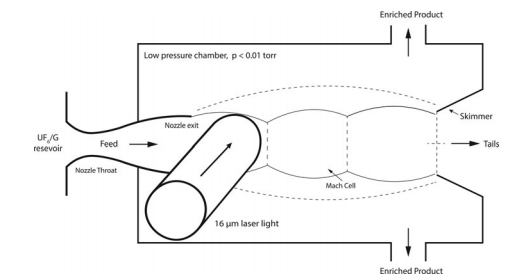
Atomic Vapor Laser Isotope Separation (AVLIS):
- Preparation: Uranium is vaporized to create a gaseous form, typically in a vacuum chamber.
- Laser Excitation: A tuned laser beam is directed at the uranium vapor. The laser's frequency is adjusted to selectively ionize or excite the U-235 atoms without affecting U-238 atoms.
- Electromagnetic Separation: The ionized U-235 atoms are then deflected using an electromagnetic field, separating them from the un-ionized U-238 atoms.
- Collection: The separated U-235 atoms are collected on a substrate for later processing, while the U-238 atoms are also collected for potential reuse or storage.
Molecular Laser Isotope Separation (MLIS):
- Preparation: Uranium hexafluoride (UF6) is used instead of vaporized metallic uranium.
- Laser Excitation: A laser selectively excites the UF6 molecules containing the U-235 isotope.
- Chemical Reaction: The excited molecules are more reactive and readily undergo a chemical reaction to form a different compound that can be easily separated from unexcited UF6 molecules.
- Collection and Conversion: The new compound is then separated from the mixture and converted back to UF6 or another form of uranium, now enriched in U-235.
What is Radiation? Can We Increase Or Reduce It?
Radioactivity is a process where unstable atoms break down and release energy in the form of particles or electromagnetic waves. This happens because these atoms have too much energy and need to move to a more stable state. When they break down, they can emit three main types of radiation: alpha particles, beta particles, and gamma rays.
Alpha particles are heavy and don't penetrate deeply, but if alpha-emitting material is ingested, inhaled, or enters the body through a wound, it can cause severe damage to the cells it interacts with.
Beta particles are lighter than alpha particles, and can penetrate further but are still mostly an issue if the radioactive material is taken into the body.
Gamma rays are electromagnetic waves which can penetrate deeply into the body and can be very damaging to internal organs.
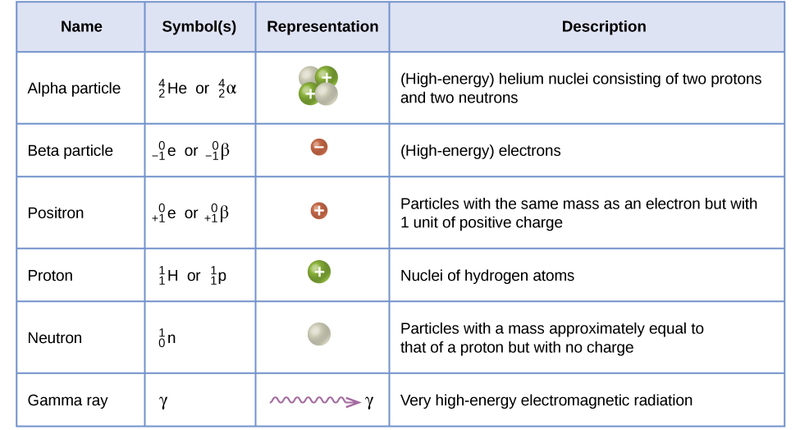
Some radioactive materials take a long time to break down, meaning they can contaminate water, soil, and air for extended periods - often tens of thousands of years. This makes cleanup difficult and costly. Once released, it's difficult to contain radiation, and it can spread easily through air, water, and food chains. Even low doses of radiation can accumulate over time and increase the risk of adverse health effects.
Radiation can directly hit the DNA inside cells, causing breaks or mutations. This can result in cells dying or becoming cancerous. When radiation interacts with water molecules in our body, it can create highly reactive free radicals. These can then damage other molecules, including DNA, proteins, and cell membranes. At extremely high doses, radiation can disrupt the nervous system and cause immediate death.
The "Salted" Cobalt-59 Bomb
Radiological qualities can be weaponised. In 1950, Manhattan Project physicist Leo Szilard engaged in a horrific thought experiment about a theoretical Doomsday weapon: the Salted Bomb.
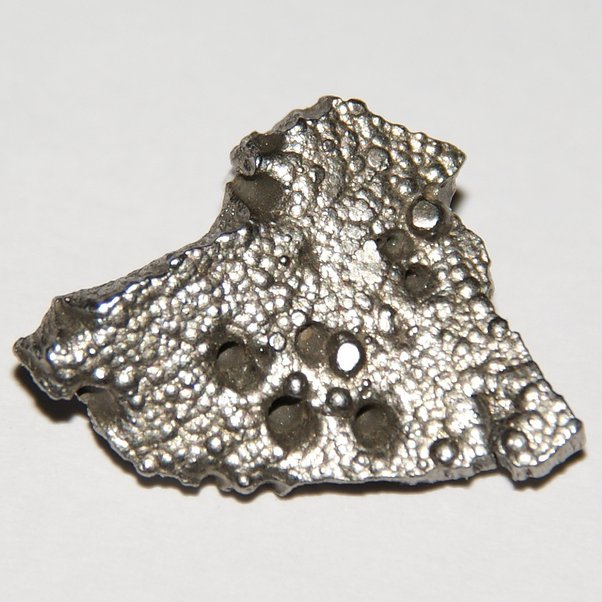
From: https://www.wondriumdaily.com/the-cobalt-bomb-leo-szilards-grim-thought-experiment/
During a round-table discussion [on a University of Chicago Round Table radio program], Szilard proposed that wiping human society off the map might just be possible if the radioactive fallout from hydrogen bombs was not thought of as an unfortunate side effect, but instead as a feature of the weapon itself.
This is because the neutron flux—free neutrons released during a nuclear explosion, could convert the cobalt-59 into cobalt-60 through neutron capture.
A giant cloud of cobalt-60 fallout would pose a major health risk to everyone on the planet. This is owing to the fact that cobalt-60 undergoes a beta decay with accompanying gamma radiation, to form the stable isotope, nickel-60.
All your nightmares about the 50-MT existence-ending weapon can be found here: https://en.wikipedia.org/wiki/Salted_bomb
The Neutron Bomb
Seven years later, Samuel T. Cohen of the Lawrence Livermore National Laboratory theorised a weapon which killed through radiation rather than the physical blast. It introduced the idea of removing the uranium casing from a hydrogen bomb to allow neutrons to travel great distances and penetrate even heavily shielded armor and structures. It was named the Enhanced Radiation Warhead (ERW), or the Neutron Bomb.
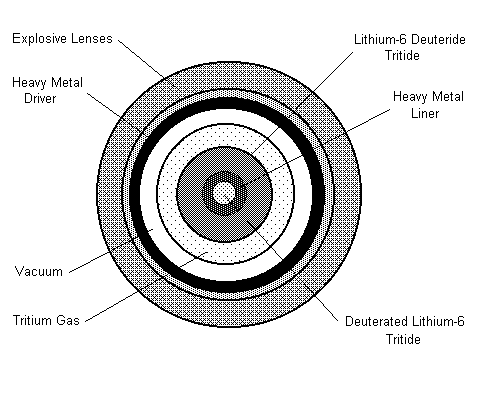
According to https://www.airandspaceforces.com/article/the-neutron-bomb/
The enhanced radiation warhead was a modification of the hydrogen or thermonuclear bomb. Like all hydrogen (or “fusion”) devices, it used a small atomic (or “fission”) bomb as a trigger to set off the hydrogen chain reaction.
The neutron bomb would release more of its energy in the form of lethal radiation. Physical damage would be limited to a relatively tight area while the radiation reached further out to penetrate Warsaw Pact armor, which was shielded against nuclear blast and heat. Since the neutron bomb produced little or no radioactive fallout or residual radiation, the target area could be reoccupied within a matter of hours.
More: https://en.wikipedia.org/wiki/Neutron_bomb
How Do We Achieve Fusion?
Fusion weapons, also known as hydrogen bombs or thermonuclear weapons, use a fission bomb as a trigger to initiate the fusion process. The Sun, basically.
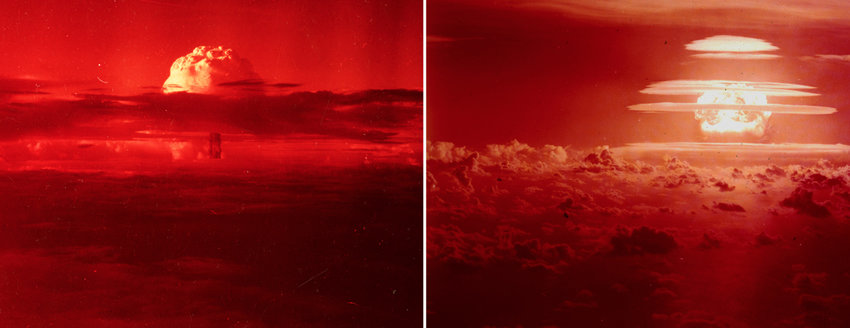
The United States initiated its hydrogen bomb project ("Operation Ivy") in the early 1950s. Physicists Edward Teller and Stanislaw Ulam were instrumental in developing the concept of using a fission bomb to trigger a fusion reaction. The idea was superficially simple: Use the radiation of an exploding fission bomb (the “primary”) to compress a special capsule that contained both fusionable and fissionable materials (the “secondary”).
The first successful test, codenamed "Ivy Mike," ("Mike" stood for "megaton") was conducted on November 1, 1952, at Enewetak Atoll in the Marshall Islands. The explosion had a yield of 10.4 megatons, significantly more powerful than any fission bomb.
The Soviet Union followed suit, led by physicist Andrei Sakharov. Their first true H-bomb test, known as the "Tsar Bomba," took place on October 30, 1961, with a yield of about 50 megatons, making it the largest nuclear explosion ever.
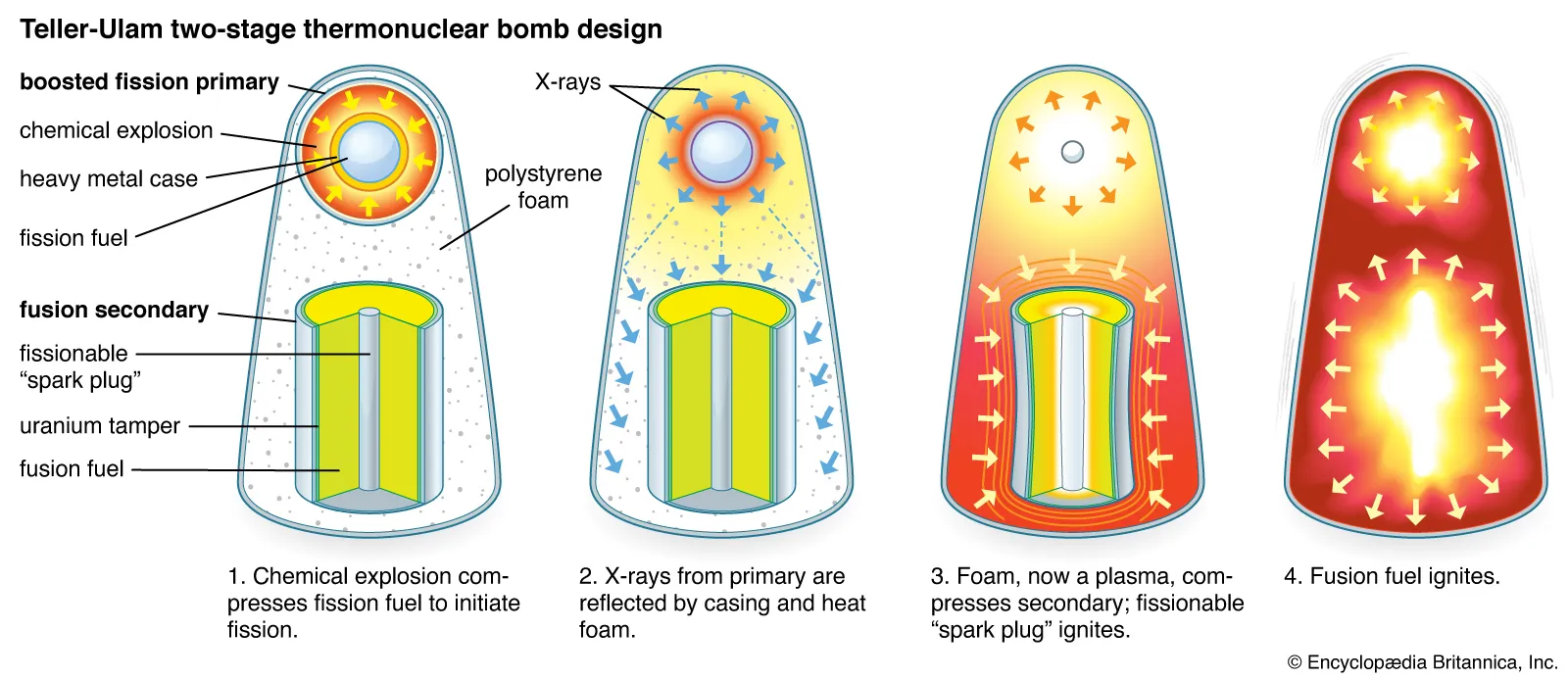
This initial fission explosion is used to compress and heat a separate part of the weapon containing fusion fuel, usually isotopes of hydrogen like deuterium and tritium. Under the extreme conditions produced by the fission bomb, the hydrogen nuclei fuse together to form helium, releasing an enormous amount of energy.
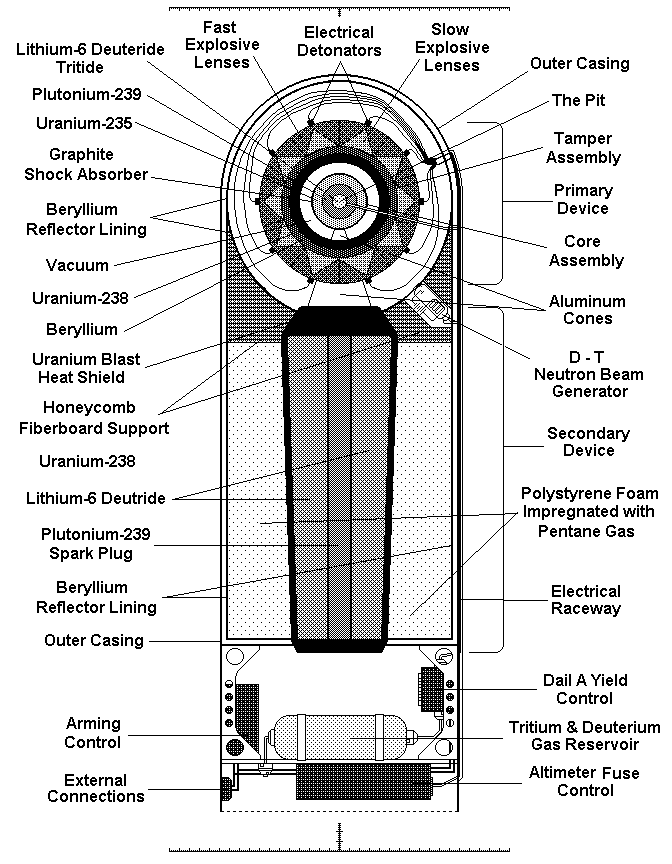
Fusion reactions produce high-energy neutrons that can initiate further fission reactions in surrounding material, creating even more energy. This can be thought of as a secondary fission process, further boosting the overall yield of the weapon. In many designs, X-rays from the initial fission explosion are channeled to compress the fusion fuel, a process known as radiation implosion. This is usually achieved using specialized materials and geometries that focus the radiation onto the fusion fuel.
Just as fission weapons need to achieve a "critical mass" for a chain reaction to occur, fusion fuel needs to reach a critical density and temperature for fusion to take place. The fission bomb effectively provides the conditions for this to happen.
Unlike fission weapons, which rely on a chain reaction, fusion reactions in a thermonuclear weapon are more of a bulk process. Once conditions for fusion are met, most of the fusion fuel undergoes fusion almost simultaneously.
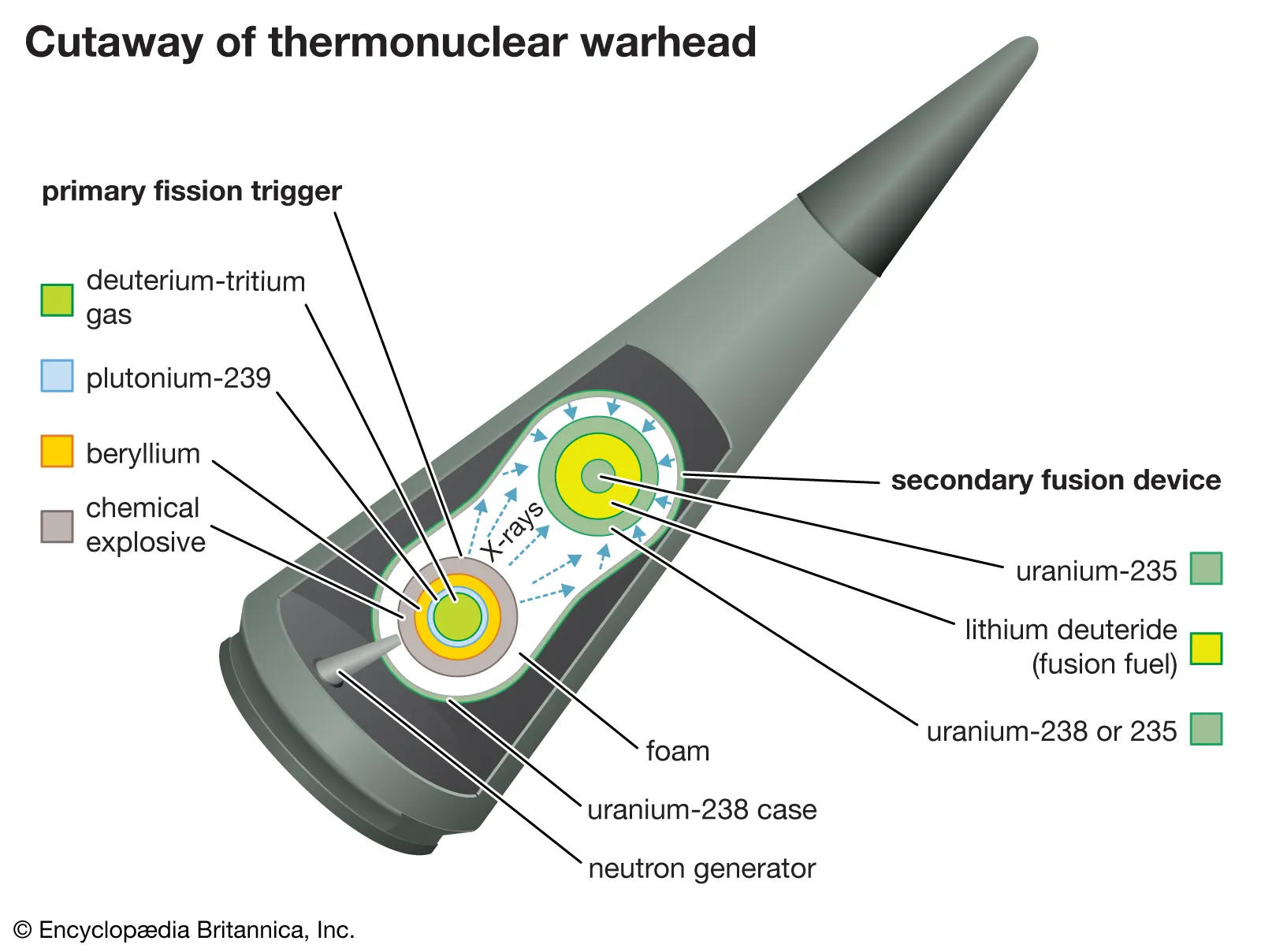
Ivy King, the second detonation of the H-Bomb program, brings home the truly terrifying scale of these weapons:
Is There Any Limit?
If igniting the atmosphere or destroying all life on earth weren't enough, some scientists wanted to go further and produce planet-destroying GIGATON weapons.
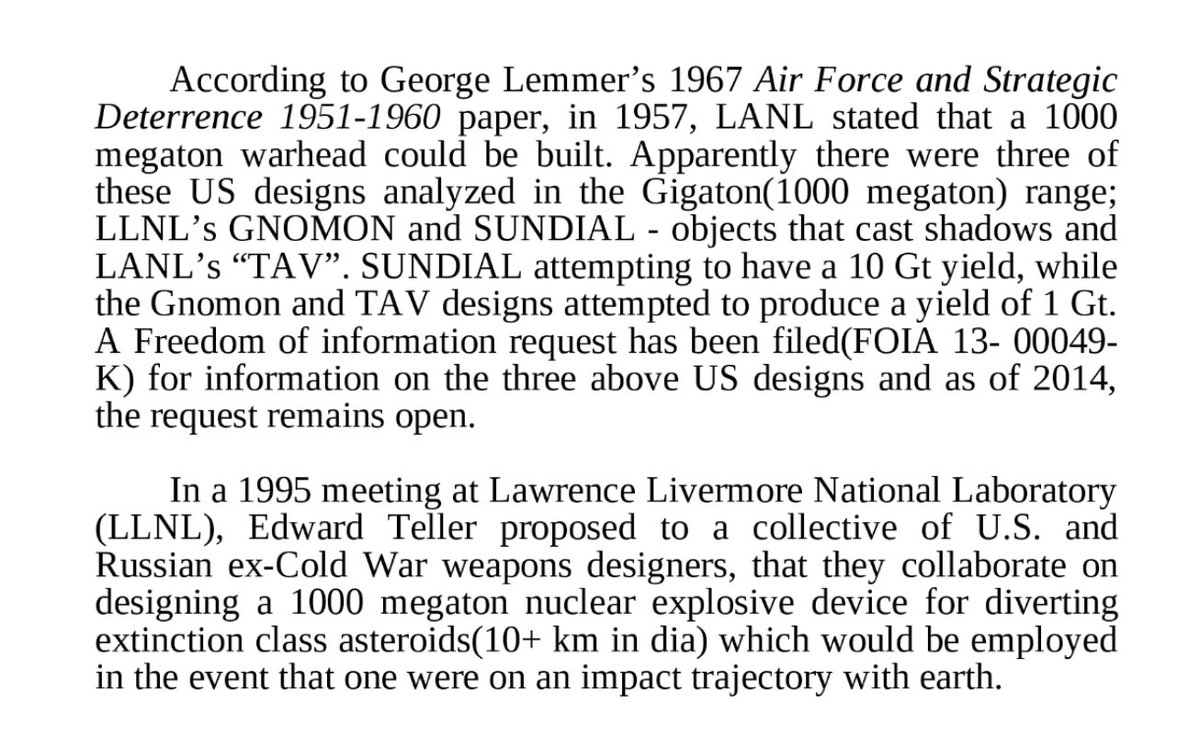
One account sticks out, detailed in a brilliant way by The Bulletin (https://thebulletin.org/2021/11/the-untold-story-of-the-worlds-biggest-nuclear-bomb/)
Only a few months later, in July 1954, Teller made it clear he thought 15 megatons was child’s play. At a secret meeting of the General Advisory Committee of the Atomic Energy Commission, Teller broached, as he put it, “the possibility of much bigger bangs.”
At his Livermore laboratory, he reported, they were working on two new weapon designs, dubbed Gnomon and Sundial. Gnomon would be 1,000 megatons and would be used like a “primary” to set off Sundial, which would be 10,000 megatons. Most of Teller’s testimony remains classified to this day, but other scientists at the meeting recorded, after Teller had left, that they were “shocked” by his proposal. “It would contaminate the Earth,” one suggested. Physicist I. I. Rabi, by then an experienced Teller skeptic, suggested it was probably just an “advertising stunt.”
This weapon could destroy entire countries.
A study from 1963 suggested that, if detonated 28 miles (45 kilometers) above the surface of the Earth, a 10,000-megaton weapon could set fires over an area 500 miles (800 kilometers) in diameter. Which is to say, an area about the size of France.
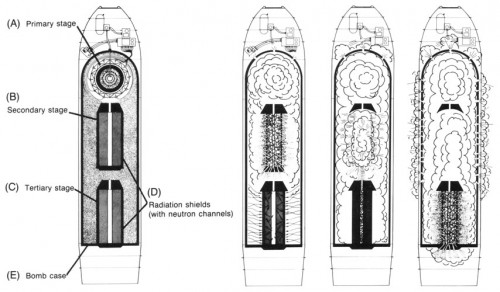
The Teller-Ulam design allows bombs to be linked together in a series. John Wheeler (American theoretical physicist on the bomb projects) apparently dubbed this the “sausage” model in Ivy Mike. Ted Taylor (another theoretical physicist) recounted that from very early on, it was clear one could have theoretically link “an infinite number” of sub-bombs connected to make one giant bomb.
The original article ("In search of a bigger boom") was published by Alex Wellerstein on his blog "Restricted Data" where a lot of comments question its viability: https://blog.nuclearsecrecy.com/2012/09/12/in-search-of-a-bigger-boom/
The 10GT weapon really would have been BACKYARD. At 6MT/tonne (the upper bound for thermonuclear weapons) it would have weighed 1667 tonnes. Even the smaller 1GT would be 167 tonnes which might have been ship or sub transportable.
For the curious at least NUKEMAP can do the effects scaling for a 10,000,000kT bomb in Washington, DC. The fireball would yield up 75% of it’s energy in about 280 seconds or so. That’s 5 minutes of 12 mile diameter fireball glow.
Which begs the question, why is it that every time scientists are faced with the inevitable dilemma of possibly destroying the entire species... out of the two options (yes/no), they always choose, "yes"?