A Sci-Fi Future Of Small Modular Liquid Fluoride Thorium Molten Salt Reactors
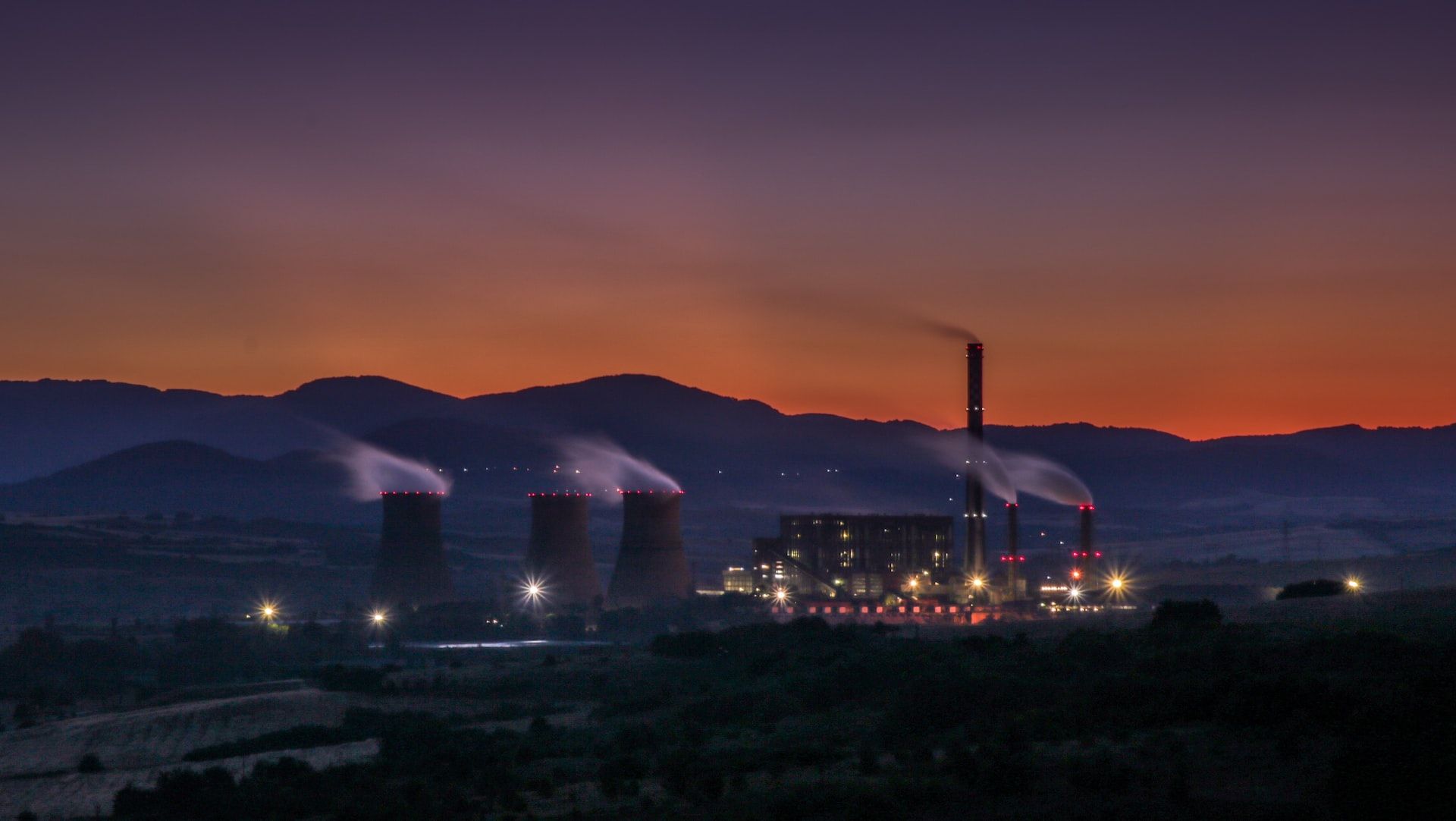
We are paying the price of the 2022 energy crisis for the failures of our politicians 30 years ago. There is no way to provide for humanity's electricity needs other than a massive and unprecedented investment in nuclear energy. There simply is no other non-hydrocarbon source which can reliably serve the demand. Anyone who claims so-called "green" sources - which ruin the landscape - can come close, is unaware of the numbers and/or can't do arithmetic.
Additionally, there is no way to store the electricity for EVs without mining fifty tonnes of lithium ore per battery, meaning the only type of viable non-hydrocarbon fuel will be liquid hydrogen. But that's for a future article.
How Much Energy Does The UK Need And Where Does It Come From?
On average, the UK demand with a population of 70 million people is around 40GW. 55% of that comes from fossil fuels (Coal 1.3GW, Gas 21GW); 27% of from renewables (10GW wind, 0.3GW hydroelectric); Nuclear comprises 5GW, biomass 2.3GW, and 0.15 classified as "other".
So in essence, our energy broadly comes from natural gas, and wind.
To put this into perspective, the US has a generation capacity of 1115GW. China has 1940GW. The UK has less than 3% of either country.
The largest nuclear power plant in the world is Kori Nuclear Power Plant in South Korea, which generates 7.4GW. The largest in the US is the Palo Verde Nuclear Generating Station in Arizona, producing 3.3GW. The largest plant in the world of any kind is the Three Gorges Dam in China, a hydroelectric facility which generates 22.5GW.
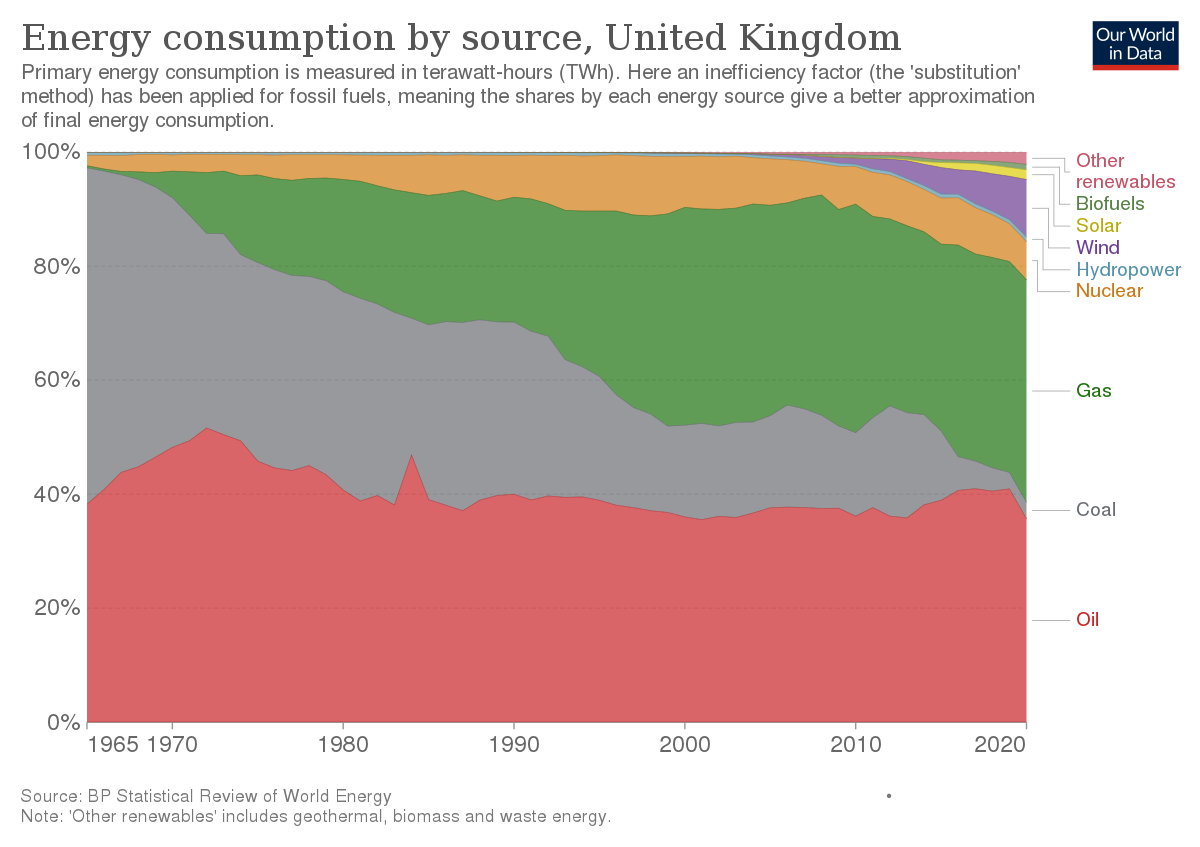
In 2019, the electricity sector's grid supply for the United Kingdom came from 43% fossil fueled power (almost all from natural gas), 48.5% zero-carbon power (including 16% nuclear power and 26.5% from wind, solar and hydroelectricity), and 8% imports.
There are 9 operational nuclear reactors at five locations in the UK generating 16% of the country's energy needs. 8 are advanced gas-cooled reactors (AGR) and one is a pressurised water reactor (PWR): https://assets.publishing.service.gov.uk/government/uploads/system/uploads/attachment_data/file/48352/1138-map-nuclear-power-stations-uk.pdf
"21% of our electricity comes from nuclear reactors, in which uranium atoms are split up to produce heat using a process known as fission. The UK’s nuclear power stations will close gradually over the next decade or so, with all but one expected to stop running by 2035."
https://www.energy-uk.org.uk/our-work/generation/electricity-generation.html
A simple explanation is available here: https://grid.iamkate.com/
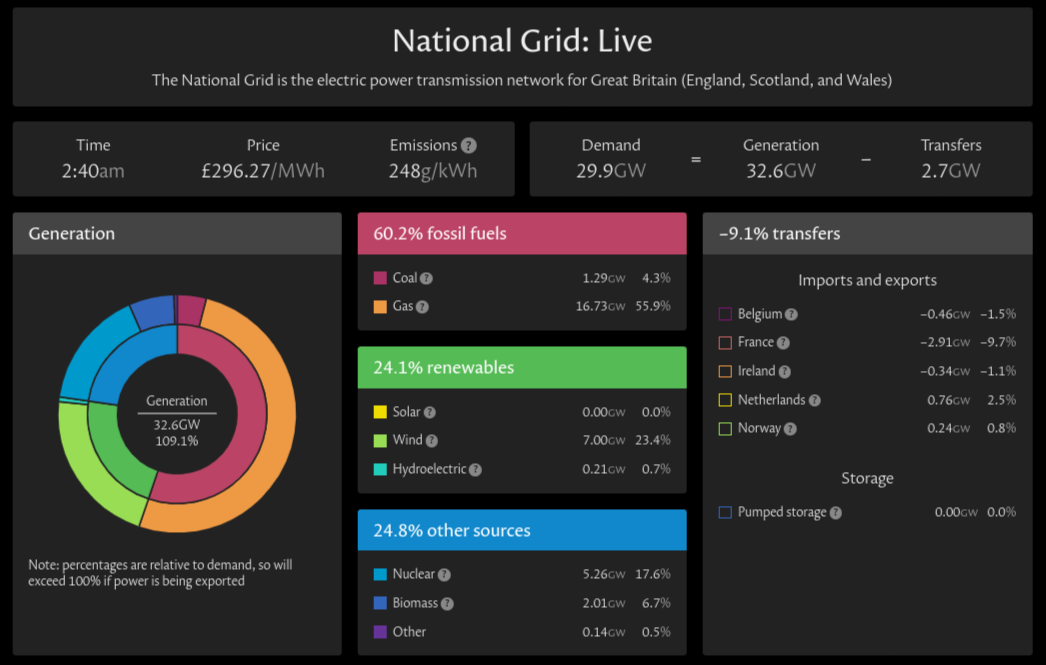
By comparison, France has 56 nuclear reactors which can generate 61 Gw, or 70% of its electricity supply.
The country needs to generate a minimum of 50GW of energy per day. If we want to supplant fossil fuels (and therefore reliance on imports, always a good idea), we need to generate 25GW from somewhere, and that doesn't account for future needs.
We cannot do with wind; nor can we do it with solar. Perhaps we might work towards it with geothermal, hydrogen, and hydro. Diversity of sources is a good idea for ensuring reliability and localisation. There's only one option: nuclear. All governments have agreed for decades.
The government is currently on the path to shutting all of its nuclear power stations barr one at Hinkley Point C, capable of 0.3GW (3,260 MWe). The graft has taken the cost to 22 billion. EDF says it plans to develop four new sites.
No new reactors have been built since Sizewell B in 1995.
This is utter madness.
How Much Is Generated By What?
Although sizes differ, we can get a look at the efficiency of specific fuel sources fairly easily by consulting the International Energy Agency: https://www.iea.org/data-and-statistics .
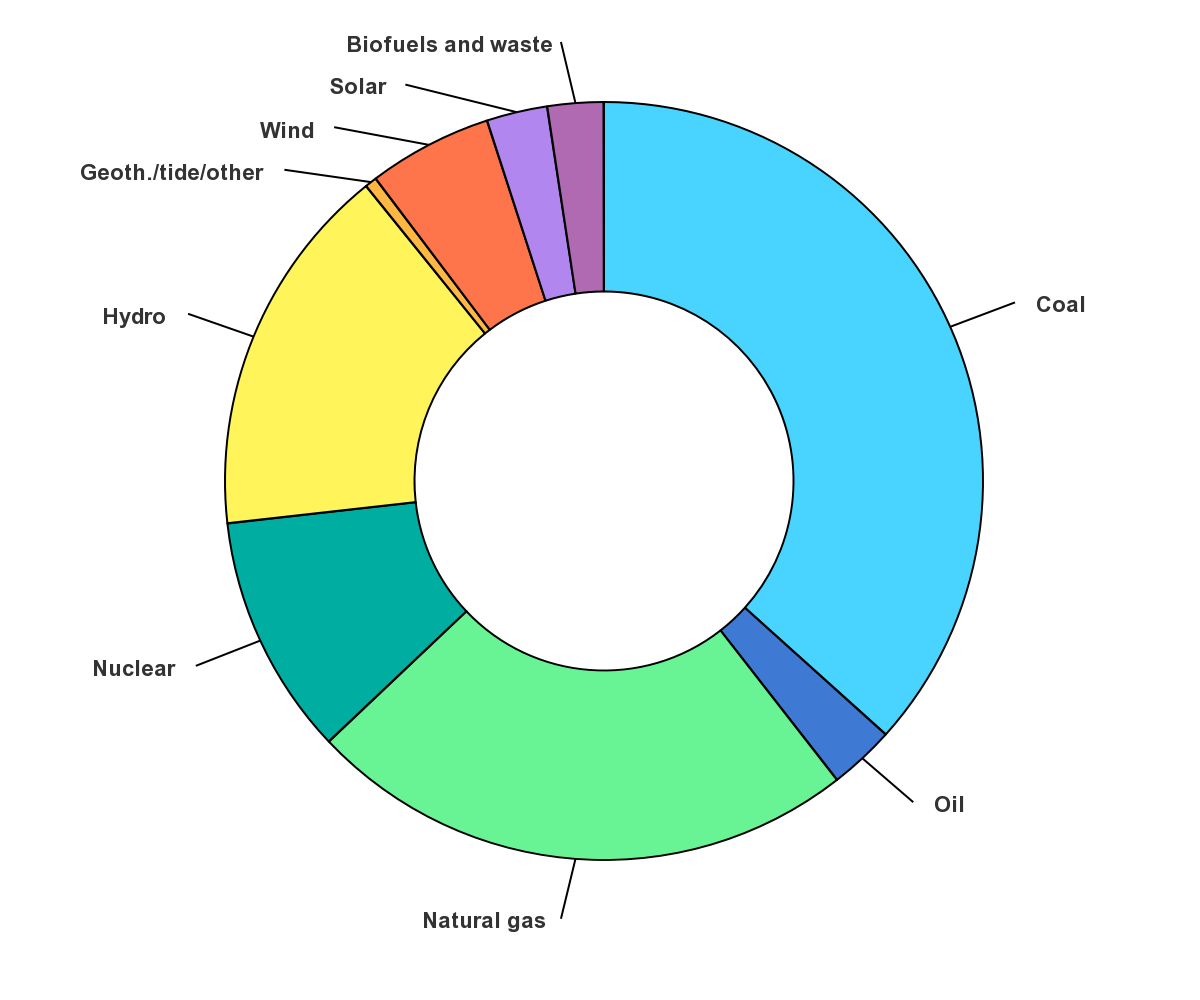
In 2019, total worldwide electricity production was nearly 27,044 TWh (26,823,200 GWh). 64.5% is fossil fuels, 10.2% is nuclear, and 25.3% is renewable.
If you think hippy ideas like solar, wind, tidal, and biomass (poop) can replace this, you are dreaming.
There are around 62,500 power plants around the world with a capacity of 6TW: https://www.washingtonpost.com/news/wonk/wp/2012/12/08/all-of-the-worlds-power-plants-in-one-handy-map/
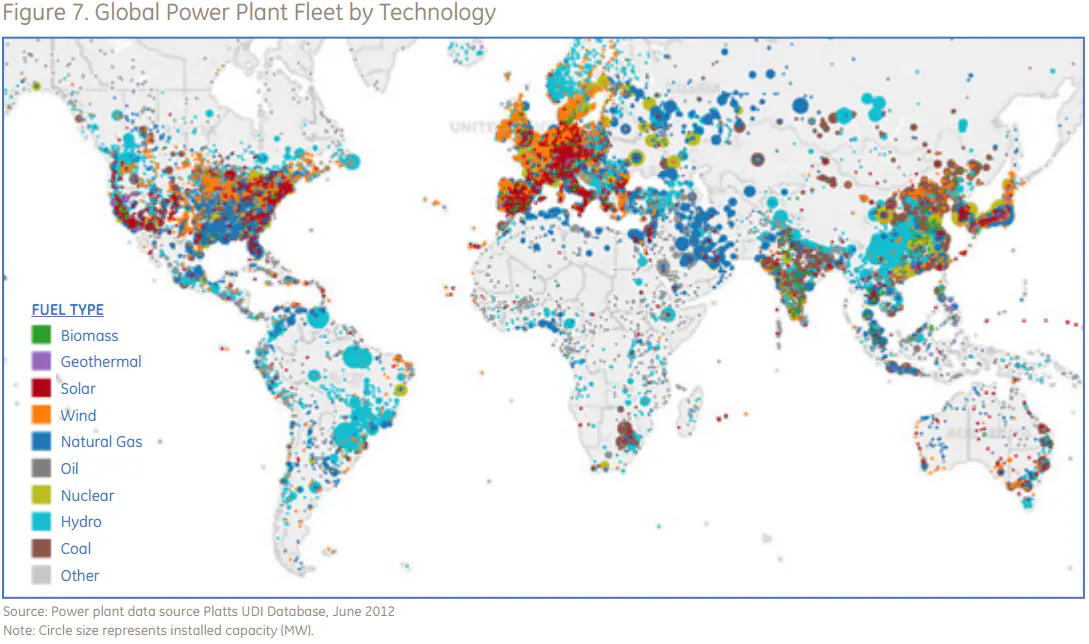
- Coal generates around 37% of the world's electricity. There are 8,500 stations generating 2TW.
- Natural gas generates around 24%. 7,320 are operating to produce 1.8TW.
- Hydroelectric facilities generate 16% or 1.3TW. 21,387 of them are in Europe.
- Nuclear reactors power around 10% of global electricity. 437 operating cores generate 0.39TW.
- Wind generates 5%. There are 341,000 turbines able to generate 0.74TW.
- Solar brings up the rear, with 92.7 billion panels powering 0.5TW.
- Other sources are biomass (143.4GW), geothermal (15.4GW), tidal (120GW), and oil.
Hydroelectric is far and away the most powerful source of energy, and comprises 70%+ of all renewable sources. The Three Gorges Dam in China generates 0.2TW on its own. The US has 2.300 hydroelectric dams generating 80GW of power.
A single solar panel generates 240 - 400 W. 10 billion 350W solar panels comprising 21,913 square miles would be needed to generate the electric needed in the US, which is an area the size of West Virginia.
An individual wind turbine can generate 2-8 MW.
For the world to become free of fossil fuels, it needs to replace - at a minimum, given a growing population - around 4TW (4000 GW), or 60%, of its power sources. Almost all the world's power consumption is in China, India, and the US.
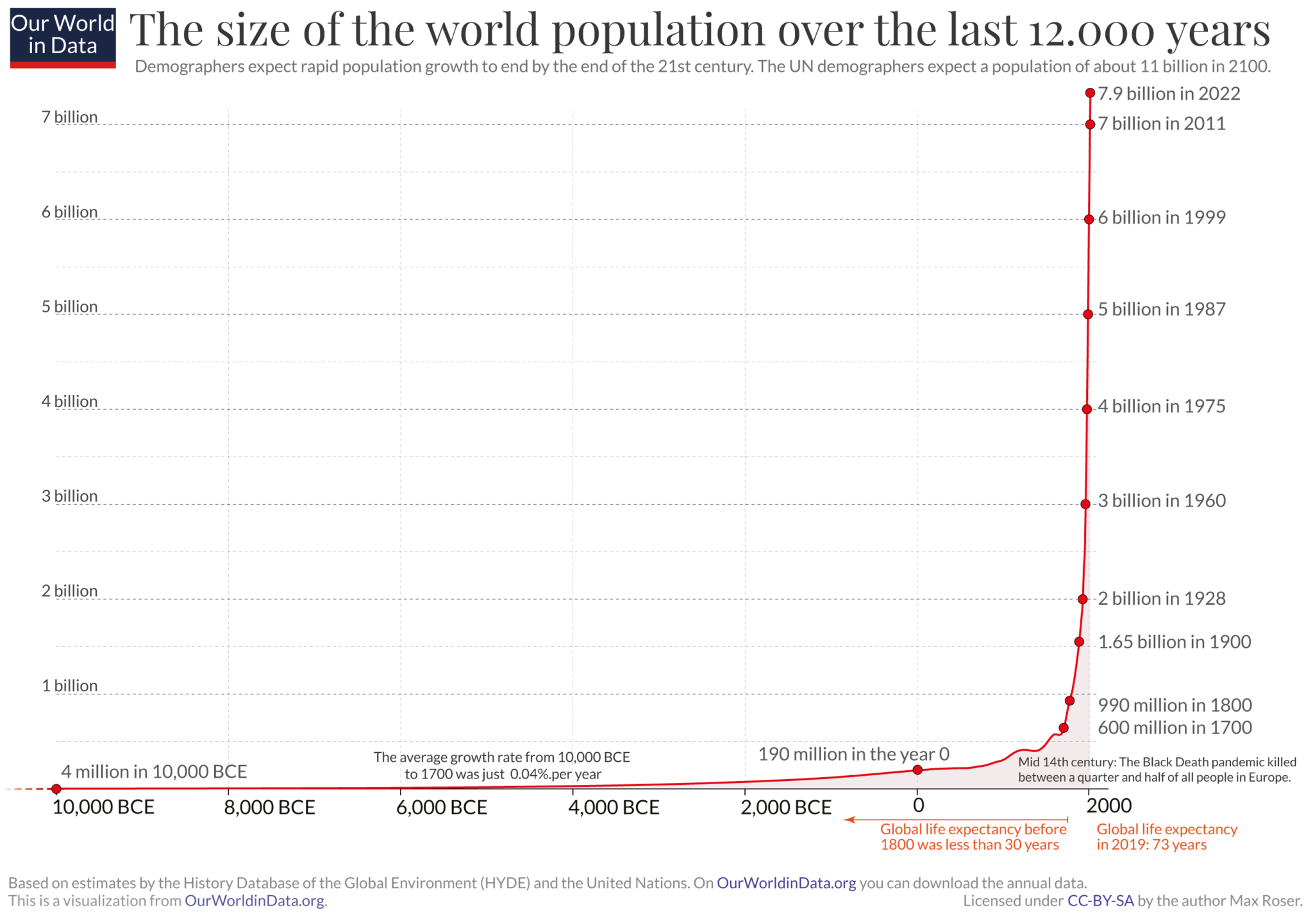
What happens if the power consumption doubles over the next twenty years, as the global population did between 1950 and 1987?
- You can't install a dam in Barbados.
- You can't put a nuclear reactor in Zimbabwe.
- You can't put geothermal or tidal in the Sahara.
- You can't put solar panels above 50° or below -50° latitude.
If the world's largest nuclear power plant can produce 7.5GW, we would need to build 533 plants (4000GW / 7.5GW) of the same size to serve the current demand, not just the future demand. Each takes 15-20 years and if we average a cost of $20B per station (10 reactors), would need $10.6 trillion.
The world consumes 5.3 million barrels of oil a day, and 22.7 TW of electricity per hour. 6.5TW of that is China. 4TW of is the US. More: https://ourworldindata.org/electricity-mix
There's no two ways about it: the UN's ideas of "net zero" by 2050 are preposterous.
The UK needs 40GW, total. Or ordinary power stations generating 3.3GW for a cost of 240BN.
What Went Wrong With UK Nuclear?
Two words: money, and fuckery.
The world's first commercial nuclear reactor was started up in the UK at Calder Hall in 1956 after a successful prototype in 1947 at Atomic Energy Research Establishment (AERE) Harwell.
The tl;dr version is the energy sector was privatised in 1988 and development stalled. The incoming Labour government at the end of the decade set the Civil Service on a path to renewable energy instead, citing the increasing problems of managing nuclear waste.
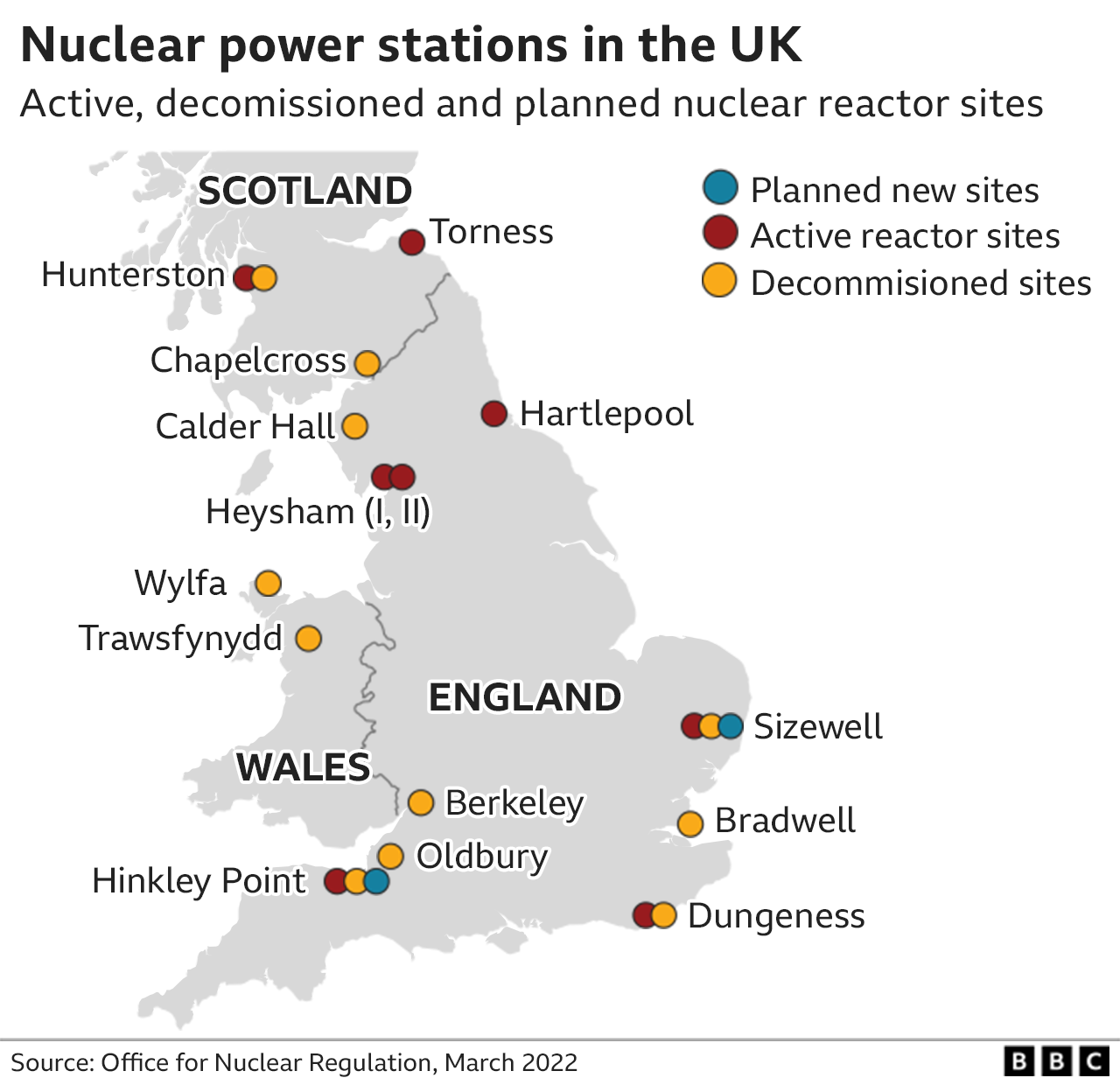
"Review of the Future Prospects for Nuclear Power in the UK" (published in May 1995), concluded that moving as much of the nuclear generating industry as possible into the private sector would bring benefits for the industry, electricity consumers and taxpayers. This led to the establishment of British Energy in 1995 to take on the more modern plants for privatization.However, the 1994 review also took the view that public sector support for a new nuclear station was inappropriate. This led to Nuclear Electric's decision not to proceed with plans to construct Hinkley Point C (which had received planning permission in 1990) and to withdraw the planning application for Sizewell C.
https://www.world-nuclear.org/information-library/country-profiles/countries-t-z/appendices/nuclear-development-in-the-united-kingdom.aspx
The bottom line is nuclear power needs heavy government investment. Taking it away killed the future industry.
Although politicians declared their intent to renew nuclear generation, they were strongly hindered by the Civil Service (i.e. Sustainable Development Commission, environment audit committee) and a Greenpeace lawsuit. The go-ahead was finally given in 2008: https://www.theguardian.com/environment/2013/oct/21/nuclear-power-in-the-uk-a-history
It's been privatised for 30+ years, and lots of people have tried. Put simply, there's no money and a tonne of activists pulling any attempt into the mud. Each plant costs £4.8 billion and replacing the existing ten amounts to £48 billion when decommissioning is factored in. British Energy is now owned by the French company EDF, who don't want to invest.
The Welsh government's support for nuclear power is patchy, at best. And their activists are virulently against it.
There is no real national debate developing around whether we want to revisit this most controversial of technologies in Wales, only a decade after the original Wylfa shut down. The only major Welsh party leader to ring a note of caution has been Plaid Cymru’s Adam Price who has said that nuclear would be the “wrong answer” and that renewables should be the way forward.
https://nation.cymru/opinion/new-nuclear-power-stations-in-wales-would-have-a-big-impact-on-our-constitutional-future-so-why-the-lack-of-debate/
The Scottish government is against nuclear power. Of course it is.
Scottish ministers have rejected new nuclear power stations in their response to the UK consultation on nuclear power. New plants were described as “dangerous and unnecessary” by the Scottish government, which previously opted out of the separate Westminster consultation pursuing deep disposal of nuclear waste.
https://www.neimagazine.com/news/newsscottish-government-rejects-new-nuclear
Then, of course, the endless line of ex-CDN lunatic left-wing groups who want the whole population to be vegan and for the sun god to power all our machinery. The less said, the better.
After that, a whole slew of left-leaning NGOs and polling companies claiming public opinion is generally against it. Best to fold them in with the Civil Service.
Why Are People Frightened Of Nuclear Power?
Three large historic nuclear accidents (Harrisburg, Chernobyl, Fukushima) have shown that the major risk during and after nuclear accidents lies in the spreading of radioactive iodine, caesium and strontium. These components are the product of the nuclear reaction and are formed in any reactor.
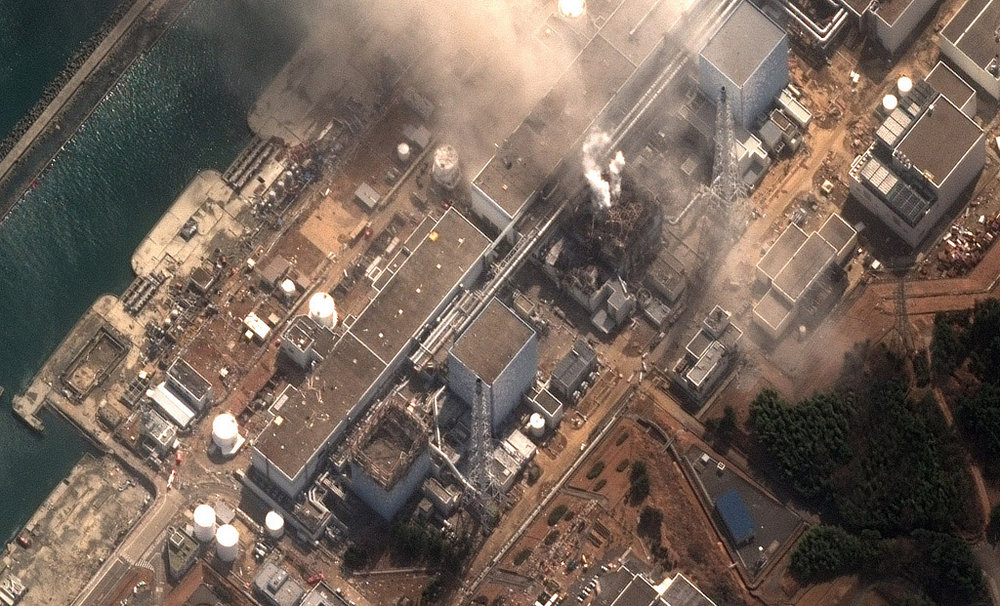
Reactors don't explode. They can melt down, and/or spread radioactivity into the atmosphere.
Meltdown occurs when the solid uranium fuel rods overheat to such an extent that the material melts, which can have dire consequences if the material then escapes its containment.
Although other radioactive elements like molybdenum, ruthenium, krypton and xenon are formed as well, iodine, caesium and strontium are the troublesome ones because in today’s light water reactors they are present in volatile form, can become airborne, and can be picked up and partly remain inside living tissue. In light water reactors in usual operation, these elements remain safely trapped inside the fuel elements and their cladding. But if an accident leads to their release, iodine and caesium may get into the atmosphere and can travel away from the reactor, over large distances.
What's The Legacy Nuclear Tech?
The UK has 8 Advanced Gas-Cooled reactors (AGR) and 1 Pressurised Water reactor (PWR). Hinkley Point C is to use the 3rd-gen European Pressurised Reactor/Evolutionary Power Reactor (PWR).
Naturally, it's a typical government farce.
EDF and Areva have been facing 'lengthy delays and steep cost overruns' on EPR reactors being built at Flamanville Nuclear Power Plant in France and at Olkiluoto Nuclear Power Plant in Finland. In October 2013, George Monbiot, a supporter of nuclear power, said that "the clunky third-generation power station chosen for Hinkley C already looks outdated, beside the promise of integral fast reactors and liquid fluoride thorium reactors. While other power stations are consuming nuclear waste (spent fuel), Hinkley will be producing it."
https://www.theguardian.com/commentisfree/2013/oct/21/farce-hinckley-nuclear-reactor-haunt-britain
In today’s uranium reactors, natural uranium is mined, purified, and chemically converted to uranium hexafluoride prior to enrichment. The process of enrichment results in two output streams, one enriched and one depleted. Approximately five parts out of six of the original uranium ends up in the depleted stream, with only one part out of six going on to be fabricated into nuclear fuel. Most of the small amount (0.7%) of the original uranium that is uranium-235 ends up in the enriched stream, but about a third remains in the depleted stream. The enriched uranium hexafluoride is chemically converted back to uranium dioxide, pressed into pellets, loaded into zirconium tubes to form fuel rods, and arranged into clusters to form fuel assemblies.
These assemblies are then loaded into a reactor where they will spend approximately five years in the core in various locations to generate nuclear energy. At the end of their useful life, they are removed from the reactor and allowed to cool in a spent fuel pond. Most of their uranium-235 has been consumed. Some of their uranium-238 has been converted into plutonium-239 and some of that has also been consumed.
Fission products have been generated from the fission of both uranium-235 and plutonium-239. Several long-lived isotopes of plutonium, americium, and curium have been formed when plutonium-239 absorbed a neutron rather than fissioned. These long-lived actinides present a disposal challenge, yet their formation at a rate that exceeds their consumption is inevitable when uranium fuel is used in a thermal-spectrum reactor of any type, including a molten-salt reactor.
How does an advanced gas-cooled reactor work?
The AGR was developed from the Magnox reactor, the UK's first-generation reactor design. The first Magnox design had been optimised for generating plutonium. It is the second generation of British gas-cooled reactors, utilizing graphite as the neutron moderator and CO2 as coolant. Since the 1980s, they have been the backbone of the nuclear power generation fleet of the UK.
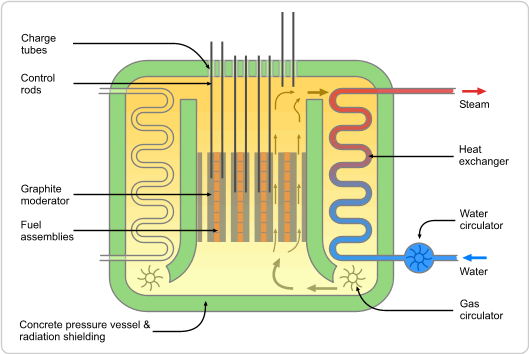
In an advanced gas-cooled (AGR) station a controlled chain reaction generates heat, which turns water into steam. The steam then powers turbines which, in turn, drive the electrical generators.
The fuel is uranium oxide pellets, enriched to 2.5-3.5%, in stainless steel tubes. The carbon dioxide circulates through the core, reaching 650°C (for improved thermal efficiency) and then past steam generator tubes outside it, but still inside the concrete and steel pressure vessel (hence ‘integral’ design). Control rods penetrate the moderator and a secondary shutdown system involves injecting nitrogen to the coolant.
It's advanced because it's the 2nd generation, and gas-cooled because it uses liquid CO2 as a coolant.
More: https://archive.uea.ac.uk/~e680/energy/energy_links/nuclear/How_an_AGR_power_station_works.pdf
How does an pressurised water reactor work?
PWRs are the majority of reactors in the world, including aircraft carriers and submarines. They were developed in the US during the fifties at Oak Ridge National Laboratory for underwater propulsion.
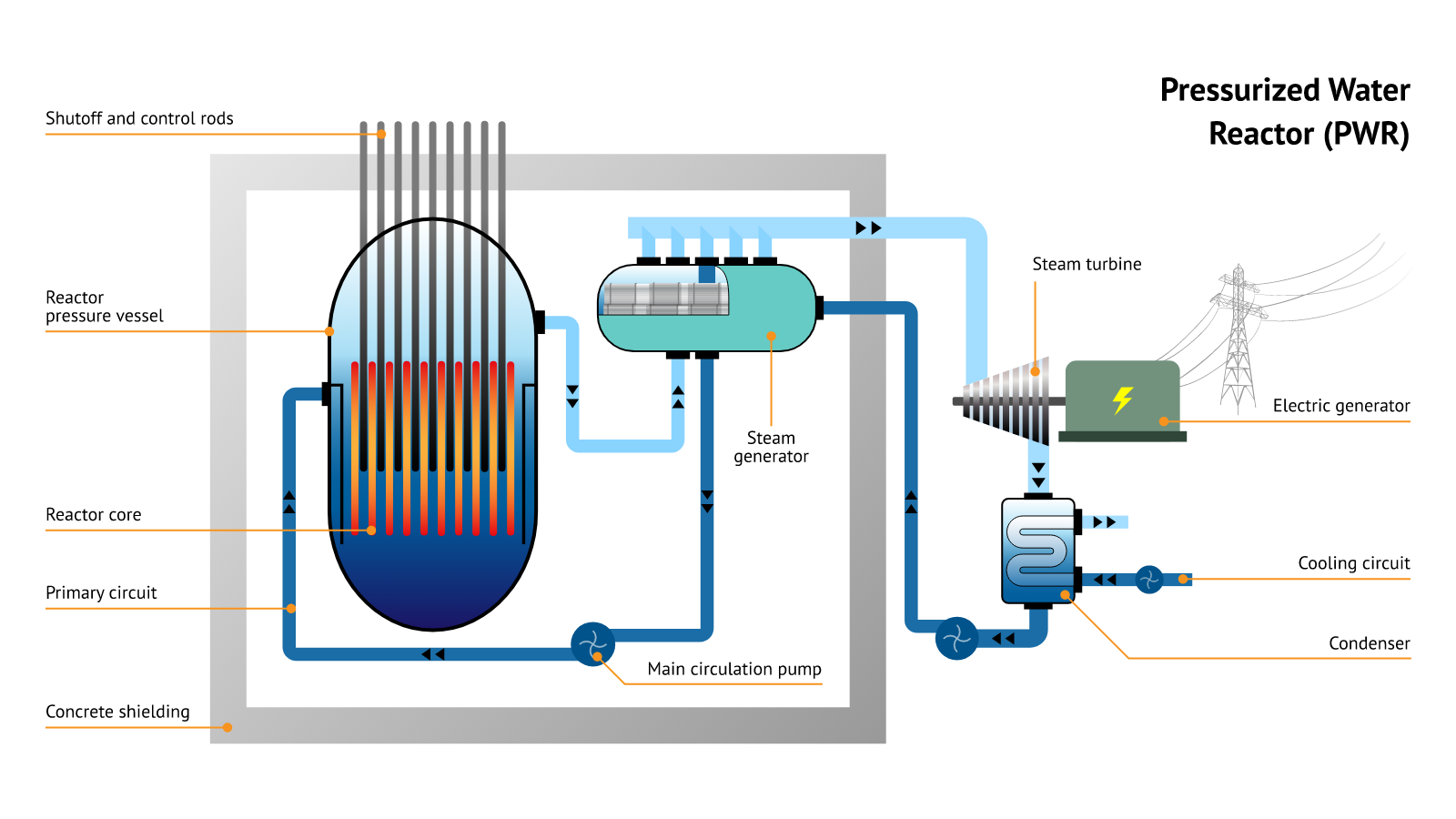
In a PWR, the primary coolant (water) is pumped under high pressure to the reactor core where it is heated by the energy released by the fission of atoms. The heated, high pressure water then flows to a steam generator, where it transfers its thermal energy to lower pressure water of a secondary system where steam is generated. The steam then drives turbines, which spin an electric generator.
According to the US NRC:
In a typical design concept of a commercial PWR, the following process occurs:
- The core inside the reactor vessel creates heat.
- Pressurized water in the primary coolant loop carries the heat to the steam generator.
- Inside the steam generator, heat from the primary coolant loop vaporizes the water in a secondary loop, producing steam.
- The steamline directs the steam to the main turbine, causing it to turn the turbine generator, which produces electricity.
The unused steam is exhausted to the condenser, where it is condensed into water. The resulting water is pumped out of the condenser with a series of pumps, reheated, and pumped back to the steam generator. The reactor's core contains fuel assemblies that are cooled by water circulated using electrically powered pumps. These pumps and other operating systems in the plant receive their power from the electrical grid. If offsite power is lost, emergency cooling water is supplied by other pumps, which can be powered by onsite diesel generators. Other safety systems, such as the containment cooling system, also need electric power. PWRs contain between 150-200 fuel assemblies.
It's also a 2nd generation design, and uses ordinary water as a coolant.
So how does an EPR work?
EPR is a third-generation PWR which first went online in China in 2018, and was developed by a coalition of two French companies and a German one: Framatome (Areva) and EDF, and Siemens.
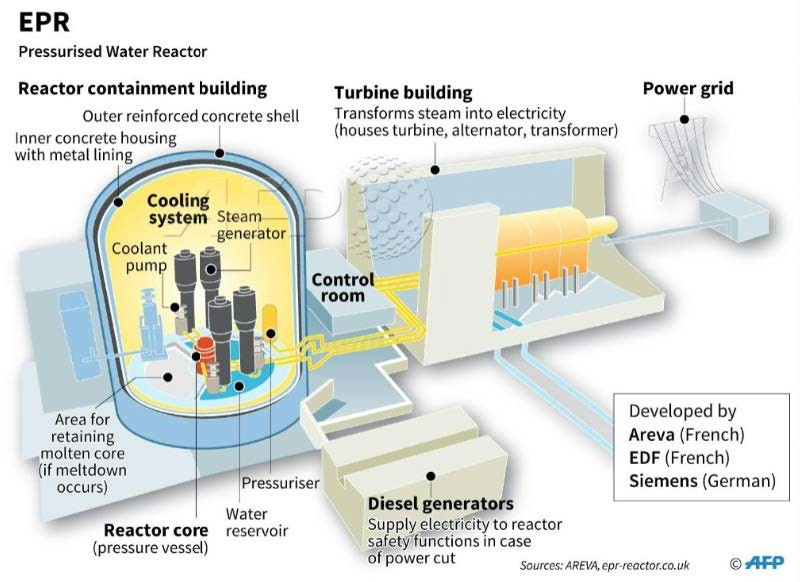
The main design goals with the EPR are based around safety.
From EDF's docs:
In a Pressurised Water Reactor (PWR) like the EPR™ reactor, ordinary (light) water is utilized to remove the heat produced inside the reactor core by nuclear fission. This water also slows down (or moderates) neutrons (constituents of atom nuclei that are released in the nuclear fission process).
Slowing down neutrons is necessary to sustain the nuclear chain reaction (neutrons have to be moderated to be able to break down the fissile atom nuclei).
The heat produced inside the reactor core is transferred to the turbine through the steam generators. Only heat is exchanged between the reactor cooling circuit (primary circuit) and the steam circuit used to feed the turbine (secondary circuit). No exchange of cooling water takes place.
The primary water is pumped through the reactor core and the primary side of the steam generators, in four parallel closed loops, by coolant pumps powered by electric motors.
Each loop is equipped with a steam generator and a coolant pump. The reactor operating pressure and temperature are such that the cooling water does not evaporate and remains in the liquid state, which increases its cooling effectiveness.
A pressuriser connected to one of the coolant loops is used to control the pressure in the primary circuit.
Feedwater entering the secondary side of the steam generators absorbs the heat transferred from the primary side and evaporates to produce saturated steam. The steam is dried in the steam generators then delivered to the turbine.
After exiting the turbine, the steam is condensed and returns as feedwater to the steam generators.
The generator, driven by the turbine, generates electricity.
http://www.epr-reactor.co.uk/scripts/ssmod/publigen/content/templates/show.asp?P=165&L=EN
What's The New Nuclear Tech?
Reactors have new configurations and are now in their 4th and 5th generations. The Generation IV International Forum (GIF), launched by the US in 2000, has 13 member countries including China, Russia, France, Japan and Britain, which have whittled down nearly 100 proffered concepts to focus research on six main nuclear reactor models. But there are plenty more.
IFR: Integral Fast Reactor
The Integral Fast Reactor (IFR), also known as Advanced Liquid-Metal Reactor, is a fast neutron reactor with a very efficient and clean nuclear fuel cycle. It was once a very active research project of the U.S. Department of Energy, and even a prototype (called Experimental Breeder Reactor-II) was build in Argonne National Laboratory. However, the project was hampered in 1994 after a series of controversies involving safety issues and nuclear non-proliferation policies.
HTGCR: High-Temperature Gas-cooled Reactor
The high-temperature gas-cooled reactor (HTGR) is fueled by a mixture of graphite and fuel-bearing microspheres. There are two competitive designs of this reactor type: (1) a German “pebble bed” system that uses spherical fuel elements, nominally 60 mm (2.5 inches) in diameter, containing a graphite-and-fuel mixture coated in a graphite shell; and (2) an American version in which the fuel is loaded into precisely located graphite hexagonal prisms that interlock to create the core of the vessel. In both variants, the coolant consists of helium pressurized to approximately 100 bars, or roughly 100 standard atmospheres.
SSTAR: Small, Sealed, Transportable, Autonomous Reactor
A small ‘fast’ reactor core in a vessel containing molten lead which both shields and cools the reactor. The vessel is essentially a self-contained cylinder that produces heat that is then converted into electricity. One of the key benefits of the concept is that the reactor does not produce extra fissile material, so it creates virtually no nuclear waste that has to be reprocessed and stored for thousands of years like with a conventional nuclear reactor.
CAESAR: Clean and Environmentally Safe Advanced Reactor
Created by Claudio Filippone, the Director of the Center for Advanced Energy Concepts at the University of Maryland, College Park and head of the ongoing CAESAR Project. The concept's key element is the use of steam as a moderator, making it a type of reduced moderation water reactor. Because the density of steam may be controlled very precisely, Filippone claims it can be used to fine-tune neutron fluxes to ensure that neutrons are moving with an optimal energy profile to split 238 92U nuclei – in other words, cause fission.
RMWR: Reduced Moderation Water Reactor
A proposed type of light water moderated nuclear power reactor, featuring some characteristics of a fast neutron reactor, thereby combining the established and proven technology of light water reactors with the desired features of fast neutron reactors. The RMWR concept builds upon the Advanced Boiling Water Reactor and is under active development in theoretical studies, particularly in Japan.
HPM: Hydrogen-moderated Self-Regulating Nuclear Power Module
A new class of nuclear power reactor using Hydrite as a neutron moderator. The design is inherently safe, as the fuel and the neutron moderator are Uranium Hydrite UH3, which is with temperature reduced to Uranium and Hydrogen. The gaseous Hydrogen exits the core, being absorbed by Hydrogen absorbing Hydrogen Storage, thus making it less critical. This means that with rising temperature the neutron moderation drops and the nuclear fission reaction in the core is dampened, leading to a lower core temperature. As more energy is taken out of the core the moderation rises, and the fission process is stoked to produce more heat.
Thorium AHWR: Advanced Heavy-Water Reactor
A vertical pressure tube type reactor cooled by boiling light water under natural circulation. The unique feature of this design is a large tank of water on top of a primary containment of vessel called gravity-driven water pool (GDWP). This reservoir is designed to perform several passive safety functions. The AHWR will be fuelled by a mix of uranium-233 and plutonium, which will be converted from thorium and uranium-238 respectively by previously deployed and domestically designed fast breeder reactors. Another version of the AHWR called AHWR-LEU will use low enriched uranium along with thorium.
Thorium KAMINI: Kalpakkam Mini Reactor
The world's only thorium-based experimental reactor at Indira Gandhi Center for Atomic Research in Kalpakkam, India. It is cooled and moderated by light water, and fueled with uranium-233 metal produced by the thorium fuel cycle harnessed by the neighbouring FBTR reactor.
FBTR: Fast Breeder Test Reactor
A 500 MWe fast breeder nuclear reactor designed to use uranium-238 to breed plutonium in a sodium-cooled fast reactor design amd presently being constructed at the Madras Atomic Power Station (MAPS) in Kalpakkam, India.
4th Gen Gas-Cooled Fast Reactor
A helium-cooled fast spectrum reactor operating within a closed fuel cycle. It combines the advantages of fast reactors, in terms of a more sustainable use of uranium resources and waste minimisation, with the wider applicability of high temperature gas reactors, in terms of high efficiency electricity generation and the co-generation of high-quality process heat.
4th Gen Lead-Cooled Fast Reactor
Lead-cooled fast reactors (LFRs) are fast spectrum reactors cooled by molten lead (or lead-based alloys) operating at high temperatures and at near atmospheric pressure, conditions enabled because of the very high boiling point of the coolant (up to 1743°C) and its low vapor pressure. Due to the fundamental thermodynamic and neutronic characteristics of lead as a coolant, LFRs offer great potential for new reactor designs that achieve a high degree of inherent safety, simplified operation, and excellent economic performance while providing the fuel material management advantages characteristic of fast reactors.
4th Gen Molten-Salt Reactor
Molten salt fission technology uses a molten salt as coolant and fuel. A nuclear reactor requires a high performance coolant for safe and efficient commercial operation. Molten salts have exceptional thermal stability, making them superior reactor coolants compared to water. This permits safe high-temperature and low-pressure reactor operation, which is crucial for heat supply to the industry, to reduce the cost of nuclear energy, and transform the efficiency of nuclear electric power generation.
4th Gen Sodium-Cooled Fast Reactor
Developed by France, Russia and China from a concept pioneered in the United States in the 1950s. A Sodium-cooled Fast Reactor (SFR) is a type of nuclear reactor that utilizes molten sodium metal as the reactor coolant as it allows for a high power density with a low coolant volume. An SFR can achieve a core power density of around 300 MW/m3 compared with Pressurized Water Reactors (PWR) that achieve 100 MW/m3. Furthermore, a sealed coolant system is needed as the sodium is highly reactive with air and water; however, the oxygen-free system prevents corrosion.
4th Gen Supercritical Water Reactor
A proposed nuclear reactor which would function above the thermodynamic critical point of water. The use of supercritical water would succeed in increasing the efficiency of the reactor to near 44%, compared to around 34% for current reactors—while potentially decreasing the capital cost by up to 30% due to smaller necessary containment and safety systems.This increase in efficiency would decrease the waste heat produced by the reactor by 35%, thereby making better use of the nuclear fuel in the reactor.
4th Gen Very-High-Temperature Reactor
Uses a graphite moderator with a once-through uranium fuel cycle. The HTGR is a type of high-temperature reactor (HTR) that can conceptually have an outlet temperature of 750 °C (1,380 °F). The reactor core can be either a "prismatic block" (reminiscent of a conventional reactor core) or a "pebble-bed" core. The high temperatures enable applications such as process heat or hydrogen production via the thermochemical sulfur–iodine cycle.
5th Gen Liquid-Core Reactor
A closed loop liquid-core nuclear reactor, where the fissile material is molten uranium or uranium solution cooled by a working gas pumped in through holes in the base of the containment vessel.
5th Gen Gas-Core Reactor
A closed loop version of the nuclear lightbulb rocket, where the fissile material is gaseous uranium hexafluoride contained in a fused silica vessel. A working gas (such as hydrogen) would flow around this vessel and absorb the UV light produced by the reaction. This reactor design could also function as a rocket engine, as featured in Harry Harrison's 1976 science-fiction novel Skyfall.
5th Gen Fission Fragment Reactor
Generates electricity by decelerating an ion beam of fission byproducts instead of using nuclear reactions to generate heat. By doing so, it bypasses the Carnot cycle and can achieve efficiencies of up to 90% instead of 40–45% attainable by efficient turbine-driven thermal reactors. The fission fragment ion beam would be passed through a magnetohydrodynamic generator to produce electricity.
Rolls-Royce & The Small Modular Reactor (UK SMR)
The press has been abuzz concerning the development of Small Modular Reactors (SMRs), which, according to RR, "occupy the footprint of two football pitches and power approximately one million homes". Each unit costs £1.8 billion and supplies approximately 0.5GW (470 MW). 16 are planned in total, with 5 going online before 2031.
The idea is one nuclear plant per city.
Rolls-Royce have been making reactors for submarines for a long time. It makes sense. The consortium comprises firms Assystem, Atkins, BAM Nuttall, Laing O’Rourke, National Nuclear Laboratory, Nuclear AMRC, Rolls-Royce, Wood and The Welding Institute.
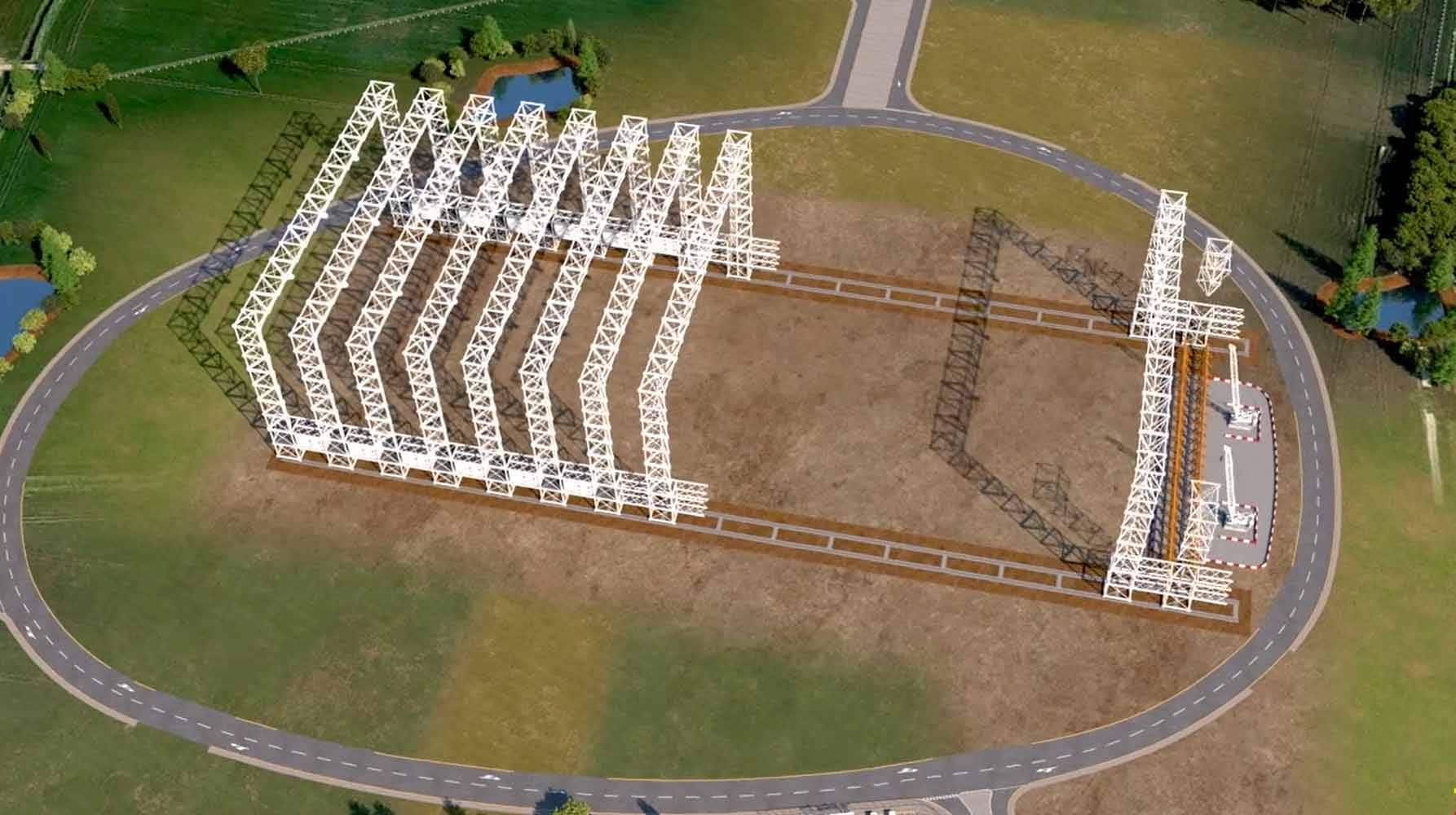
According to Leftipedia:
RR is preparing a small modular reactor (SMR) design called the UK SMR, a close-coupled three-loop pressurized water reactor (PWR) design. Power output was initially designed to be 440 MWe, and subsequently increased to 470 MWe which is above the usual range considered to be a SMR. It should be able to power a city the size of Sheffield. The intended fuel is uranium dioxide (UO2). A modular forced draft cooling tower will be used. The design targets a 500 day construction time, on a 10 acres (4 ha) site. Overall build time is expected to be four years, two years for site preparation and two years for construction and commissioning.
In November 2022 Rolls Royce announced that the sites at Trawsfynydd, Wylfa, Sellafield and Oldbury would be prioritised for assessment as potential locations for multiple SMRs.
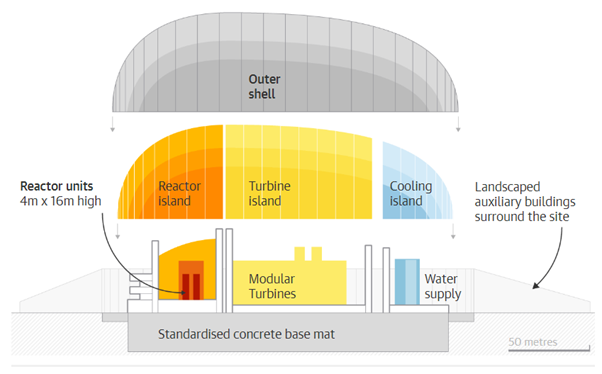
According to NeutronBytes:
It is a three loop, close-coupled, Pressurized Water Reactor (PWR) provides a power output at circa 400-450 MWe from 1200- 1350 MWth using industry standard UO2 fuel.
Coolant is circulated via three centrifugal Reactor Coolant Pumps (RCPs) to three corresponding vertical u-tube Steam Generators (SGs). The design includes multiple active and passive safety systems, each with substantial internal redundancy.
The target cost for each station is GBP1.8 billion, U$D 2.3 billion, by the time five have been built, with further savings possible, Rolls-Royce said. Each power station will be able to operate for 60 years and provide 440 Mwe of electricity.
[snip]
The U.S. Army released a report a year ago detailing the possible uses of mobile nuclear power. The ideal is a power plant system that can fit inside a standard 40-foot shipping container, can be loaded onto a military transport plane or Navy ship, and can generate up to 20 megawatts of power for 10 years or longer without resupply, according to the report.
https://neutronbytes.com/2019/11/09/rolls-royce-reveals-440-mw-commecial-reactor-design/
Five reactors means £10B for 2.5GW. 16 means £32B for 8GW. It's nowhere near enough, but it's a start. To power the whole UK, 80 units would be needed, for which the sites aren't available.
More:
- https://www.rolls-royce.com/innovation/small-modular-reactors.aspx#/
- https://www.rolls-royce-smr.com/
Others doing work in the UK include:
Westinghouse (https://www.westinghousenuclear.com/new-plants/small-modular-reactor) is developing a 225MWe pressurised water reactor, largely based on technologies deployed in its AP1000 design. The Nuclear AMRC is engaged with Westinghouse on potential UK development, and has completed a manufacturability study which showed that the UK supply chain has the capabilities to effectively manufacture the reactor pressure vessel. The study determined that Westinghouse’s use of UK advanced manufacturing techniques offers a potential 50 per cent reduction in delivery lead times and offers substantial cost savings to SMR manufacturing.
NuScale Power (http://www.nuscalepower.com/) is developing the Power Module, a 50MWe pressurised water reactor and generator, designed to be deployed in clusters of up to 12 per site. US-based NuScale is working with the Nuclear AMRC to collaborate on UK development, and is actively seeking potential UK suppliers. In July 2016, the Nuclear AMRC hosted NuScale’s UK suppliers day. NuScale has launched a supplier registration page at suppliers.nuscalepower.com
Urenco (https://urenco.com/) is leading development of an ultra-small design called U-Battery. Based on pebble bed technology, each reactor will produce just 4MWe plus 10MWt. Target markets include back-up power, desalination plants and smart cities. Urenco is working with the Nuclear AMRC to support development.
Moltex Energy (http://www.moltexenergy.com/), a privately-held UK company, is developing a 150MWe stable salt reactor, designed for modular deployment in clusters of up to 10 units per site. It is engaged with the Nuclear AMRC on potential development.
GF Nuclear (http://gfnuclear.co.uk/) is an independent power generation company which aims to develop the South Korean 100MWe Smart reactor in the UK. GF Nuclear says it will give UK engineering firms every opportunity to supply elements of the reactor’s core and other critical nuclear systems.
Not content with their total failure to date, the government is wasting even more money by setting up a prototype fusion reactor. One small problem: fusion technology doesn't exist yet.
The U.K. government has chosen the location for a prototype fusion energy plant, with the country’s Energy Secretary, Jacob Rees-Mogg, describing the technology as “a great hope.”
In a statement Monday, authorities said the STEP facility would be based at the West Burton power station, in Nottinghamshire, England. The aim is to build STEP, which stands for Spherical Tokamak for Energy Production, by 2040.
Rees-Mogg’s optimism was not shared by everyone. “It’s been a long-running joke that nuclear fusion is only a few decades away – we’ve heard this for the best part of 70 years,” Mike Childs, Friends of the Earth’s head of science, policy and research, said in a statement sent to CNBC. “So anyone suggesting that we’ll have a nuclear fusion plant producing affordable energy in the UK by 2040 is dreaming,” Childs added.
https://www.cnbc.com/2022/10/05/uk-selects-site-for-prototype-fusion-energy-plant.html
A pattern should be emerging here. Preposterous demands and promises by people who appear to be dreaming.
What's So Great About A Thorium Fuel Cycle?
Only three naturally-occurring minerals to date can be used for fission: Uranium-235, Plutonium-239, and Thorium-232 (Uranium-233). An original Thorium reactor was built in the US, but after 1973, the government settled on Uranium. It's not new. It was simply sidelined during the original decision-making of the fifties and sixties.
A good start on the subject is the Thorium Energy Alliance https://thoriumenergyalliance.com/ and/or https://energyfromthorium.com/.
Every operating nuclear reactor in existence uses Uranium oxide as its fuel. It is 40x more common than silver, and 500x more common than gold. 99.27% of it is U-238. The largest deposits of Uranium ore are found in Australia, Kazakhstan, and Canada; with the highest-grade deposits found in the Athabasca Basin region of the latter. Kazakhstan overwhelmingly dominates current annual production with 21,819 tonnes out of a total of 48,332 tonnes.
What is Thorium?
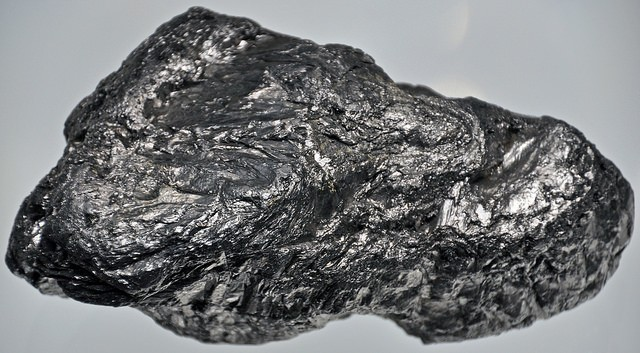
Thorium (Th, atomic number 90) was discovered in 1828 by the Norwegian amateur mineralogist Morten Thrane Esmark and identified by the Swedish chemist Jöns Jacob Berzelius, who named it after Thor, the Norse god of thunder. It is found as Th-232, an unstable isotope with a half-life of 14.05 billion years. Naturally silver, it tarnishes black when exposed to air, forming its dioxide.
Thorium is the 41st most abundant element in the Earth's crust and 2.5 - 20% of Monazite (as Thorium phosphate), the highest deposits of which are found in India, South Africa, Brazil, Australia, and Malaysia. World Monazite resources are estimated to be about 12 million tons, two-thirds of which are in heavy mineral sands deposits on the south and east coasts of India. Hence India's intense efforts and focus on Thorium reactors. Scientists estimate the crust could contain up to 120 trillion tonnes.
The distribution is as follows:
India | 980,000 | 25.1% |
Australia | 489,000 | 18.7% |
US | 400,000 | 15.3% |
Turkey | 344,000 | 13.2% |
Brazil | 302,000 | 11.6% |
Venezuela | 300,000 | 11.5% |
Norway | 132,000 | 5.1% |
Egypt | 100,000 | 3.8% |
How Does It Work?
In a nuclear power reactor, there are two types of fuel. The first is fissile material, which splits when hit by neutrons, releasing a large amount of energy and also releasing two or three new neutrons. These can split more fissile material, resulting in a continued chain reaction. Examples of fissile fuels are U-233, U-235 and Pu-239. The second type of fuel is called fertile. Examples of fertile fuel are Th-232 (mined thorium) and U-238 (mined uranium). In order to become fissile these nuclides must first absorb a neutron that's been produced in the process of fission, to become Th-233 and U-239 respectively. After two sequential beta decays, they transmute into fissile isotopes U-233 and Pu-239 respectively. This process is called breeding.
In simple terms, the source material Thorium-232 naturally converts into Uranium-233, the reactor fuel. Th-232 captures a neutron and becomes Th-233. That decays to Protactinium-233 by emitting an electron and anti-neutrino. This process repeats once more, decaying the molecule to U-233.

Or, according to Leftipedia:
In the reactor, 232 Th is transmuted into the fissile artificial uranium isotope 233 U which is the nuclear fuel. Unlike natural uranium, natural thorium contains only trace amounts of fissile material (such as 231 Th ), which are insufficient to initiate a nuclear chain reaction. Additional fissile material or another neutron source is necessary to initiate the fuel cycle. In a thorium-fuelled reactor, 232 Th absorbs neutrons to produce 233 U . This parallels the process in uranium breeder reactors whereby fertile 238 U absorbs neutrons to form fissile 239 Pu . Depending on the design of the reactor and fuel cycle, the generated 233 U either fissions in situ or is chemically separated from the used nuclear fuel and formed into new nuclear fuel.
The best and most comprehensive overview can be found at the World Nuclear Association site:
Thorium (Th-232) is not itself fissile and so is not directly usable in a thermal neutron reactor. However, it is ‘fertile’ and upon absorbing a neutron will transmute to uranium-233 (U-233), which is an excellent fissile fuel material. In this regard it is similar to uranium-238 (which transmutes to plutonium-239). All thorium fuel concepts therefore require that Th-232 is first irradiated in a reactor to provide the necessary neutron dosing to produce protactinium-233. The Pa-233 that is produced can either be chemically separated from the parent thorium fuel and the decay product U-233 then recycled into new fuel, or the U-233 may be usable ‘in-situ’ in the same fuel form, especially in molten salt reactors (MSRs).
Thorium fuels therefore need a fissile material as a ‘driver’ so that a chain reaction (and thus supply of surplus neutrons) can be maintained. The only fissile driver options are U-233, U-235 or Pu-239. (None of these is easy to supply).
https://world-nuclear.org/information-library/current-and-future-generation/thorium.aspx
Why Would You Bother?
There are a lot of reasons to use Thorium over Uranium, and few of them are about it being a "green" alternative. A useful scrutiny is here: http://www.janleenkloosterman.nl/reports/thesis_pool_2013.pdf
1. There's much more of it. 3x more than there is Uranium. The Thorium Energy Alliance estimates "there is enough thorium in the United States alone to power the country at its current energy level for over 1,000 years."
2. It's harder to weaponise its waste. Thorium is not fissile like uranium, so packed thorium nuclei will not begin to split apart and explode. Uranium-232 tends to "poison" the uranium-233.
3. There's less nuclear waste which decays faster. 500 years instead of 10,000, and 1000x less storage required.
4. It makes its own fuel. Breeding reactors produce at least as much fissile material as they consume. Non-breeding reactors, on the other hand, require additional fissile material, such as uranium-235 or plutonium to sustain the reaction.
5. No enrichment is needed. Natural Thorium can used.
6. It's far more efficient. One ton of thorium can produce as much energy as 200 tons of uranium, or 3,500,000 tons of coal.
7. The mining is less damaging. Thorium mines are open pits and it's easier to find.
8. Meltdowns aren't possible. A fusible plug at the bottom of the reactor melts in the event of a power failure or if temperatures exceed a set limit, draining the fuel into an underground tank for safe storage.
The Thorium MSR Foundation (see notes later) deduces:
A 1000MWe nuclear power plant consumes 3,2 kilograms of fission fuel per day. In case of an LWR, an average of roughly 680 kilograms of natural uranium per day needs to be mined to provide that daily consumption. In case of a thorium MSR, 3,2 kilograms of thorium per day needs to be mined to produce the same amount of energy. (Hargraves & Moir, 2010). This means that a year of electricity for a medium sized city (1 gigawatt year, roughly the amount of electricity for a city of one million inhabitants, living by western standards) can be produced with one tonne (1000 kg) of natural thorium. Another relevant comparison would be with coal. With coal, a quantity of 3,3 million tonnes (equals roughly 570 kilometres of full freight train cars) would be needed to produce the same amount of energy.
In summary, it's safer, more efficient, and there's lots of it. In "Superfuel: Thorium, the Green Energy Source for the Future", Richard Martin claims:
"Thorium could provide a clean and effectively limitless source of power while allaying all public concern—weapons proliferation, radioactive pollution, toxic waste, and fuel that is both costly and complicated to process."
Naturally, the UK has dismissed it. Because, of course it has.
For instance, in its 2010 report [NNL (2010)], the UK National Nuclear Laboratory said it believes that the thorium fuel cycle does not currently have a role to play in the UK context, other than its potential application for plutonium management in the medium to long term. The technology is innovative, although technically immature and currently not of interest to the utilities, representing significant financial investment and risk without notable benefits. In many cases, the benefits of the thorium fuel cycle have been overstated.
https://network.bellona.org/content/uploads/sites/4/Certain-issues-of-economic-prospects-of-thorium-based-nuclear-energy-systems.pdf
And The Drawbacks?
There are several, not limited to the investment capital required.
1. It can be used to produce nukes with U-233. If you adjust the process to sidestep Protactinium, you can harvest the fissile material for a weapon: https://www.popularmechanics.com/science/energy/a11907/is-the-superfuel-thorium-riskier-than-we-thought-14821644/ and https://www.tandfonline.com/doi/abs/10.13182/NT10-203
2. A lot of time, money, and commitment is required. Isn't it always?
3. Gamma rays from U-232 are a byproduct. Much more expensive radiation shielding is required.
4. Higher temperatures are required. Thorium oxide has a higher melting point than Uranium.
The Bulletin of Atomic Scientists refutes the claims made by Thorium advocates, and openly derides them: https://thebulletin.org/2014/05/thorium-the-wonder-fuel-that-wasnt/ .
So, in summary, a lot of up-front work and money, and some nasty production emissions which could theoretically be weaponised or be a health risk. But by and large, a considerable revolution of the wheel.
As should become apparent, Thorium-based power is a simple improvement and optimisation of what we already have, and its biggest problem is the marketing of it as a "miracle" or "super" fuel which can save the world.
How Does It Work Within A Molten Salt Reactor?
Now, if we have the theory of using the natural decay of Thorium to U-233 as our fuel generation cycle, the natural question is then of which containment process would best suit it. 7 types of reactors are able to use it:
- Heavy Water Reactors (PHWRs)
- High-Temperature Gas-Cooled Reactors (HTRs)
- Boiling (Light) Water Reactors (BWRs)
- Pressurised (Light) Water Reactors (PWRs)
- Fast Neutron Reactors (FNRs)
- Molten Salt Reactors (MSRs) - design stage
- Accelerator Driven Reactors (ADS) - design stage
The interesting part of the history of Thorium fuel is we have already built it. In 1959 Dr. Alvin Weinberg led the MSRE (Molten Salt Reactor Experiment) at Oak Ridge National Laboratory which operated critical for roughly 15,000 hours from 1965 to 1969. He realised it was meltdown-proof.
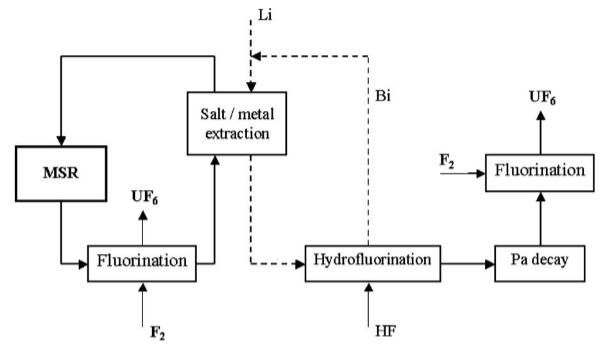
The MSRE was a 7.4 MWth test reactor simulating the neutronic "kernel" of a type of inherently safer epithermal thorium breeder reactor called the liquid fluoride thorium reactor. It primarily used two fuels: first uranium-235 and later uranium-233. The latter 233UF4 was the result of breeding from thorium in other reactors. Since this was an engineering test, the large, expensive breeding blanket of thorium salt was omitted in favor of neutron measurements.
https://en.wikipedia.org/wiki/Molten-Salt_Reactor_Experiment
https://en.wikipedia.org/wiki/Liquid_fluoride_thorium_reactor
Weinberg was forced out in 1973.
Moir and Teller explain what happened:
"The competition came down to a liquid metal fast breeder reactor (LMFBR) on the uranium-plutonium cycle and a thermal reactor on the thorium-233U cycle, the molten salt breeder reactor. The LMFBR had a larger breeding rate ... and won the competition."
Put simply, you can't make weapons out of Thorium, or weaponise it effectively. It was "the alternative path that was not taken" because military application was favoured. That's why it got sidelined.
This "two track" road is reflected in the Bulletin of Atomic Scientists:
The second track—now largely forgotten—was based on thorium-fueled reactors. This option was attractive because thorium is far more abundant than uranium and holds the potential for producing an even larger amount of uranium 233 in reactors designed specifically for that purpose. In pursuing this track, the government produced a large amount of uranium 233, mainly at weapons production reactors. Approximately two tons of uranium 233 was produced, at an estimated total cost of $5.5 to $11 billion (2012 dollars), including associated cleanup costs.
The federal government established research and development projects to demonstrate the viability of uranium 233 breeder reactors in Minnesota, Tennessee, and Pennsylvania. By 1977, however, the government abandoned pursuit of the thorium fuel cycle in favor of plutonium-fueled breeders, leading to dissent in the ranks of the AEC. Alvin Weinberg, the long-time director of the Oak Ridge National Laboratory, was, in large part, fired because of his support of thorium over plutonium fuel.
By the late 1980’s, after several failed attempts to use it commercially, the US nuclear power industry also walked away from thorium. The first commercial nuclear plant to use thorium was Indian Point Unit I, a pressurized water reactor near New York City that began operation in 1962. Attempts to recover uranium 233 from its irradiated thorium fuel were described, however, as a “financial disaster.” The last serious attempt to use thorium in a commercial reactor was at the Fort St. Vrain plant in Colorado, which closed in 1989 after 10 years and hundreds of equipment failures, leaks, and fuel failures. There were four failed commercial thorium ventures; prior agreement makes the US government responsible for their wastes.
https://thebulletin.org/2014/05/thorium-the-wonder-fuel-that-wasnt/
Energy From Thorium summarises how Liquid Fluoride Thorium Reactors (LFTRs) work:
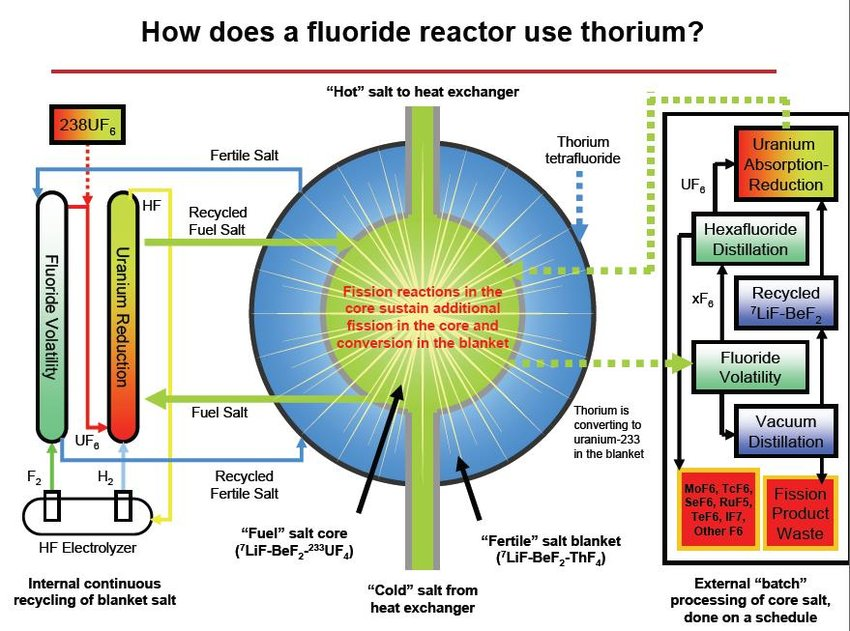
In a future liquid-fluoride thorium reactor, the fuel cycle would be quite different. The reactor would ideally be started by a modest inventory of uranium-233. Fission of U-233 in the reactor generates thermal power as well as excess neutrons that would be captured in a blanket fluid containing thorium tetrafluoride in solution. Thorium, having absorbed a neutron, first decays to protactinium and ultimately to uranium-233. New fuel would be chemically removed from the blanket fluid either at the uranium stage or the protactinium stage, which has additional complexity and advantages.
The new uranium fuel would be introduced into the fuel salt of the LFTR at the same rate at which it is consumed. Uranium-233 is consumed at high efficiency (91%) in a thermal spectrum reactor and that which is not consumed goes on to form uranium-235, which is also consumed at high efficiency (85%) in a thermal spectrum reactor. This limits considerably the amount of material that can reach the stage of the first transuranic, in this case, neptunium-237, and thus the issue of long-lived actinide waste production.
The fuel salt used in the LFTR is chemically processed as the reactor operates, removing fission products while retaining actinide fuels. This allows the creation of an actinide-free waste stream which decays to acceptable radioactivity levels in approximately 300 years, strongly governed by the 30-year half-lives of cesium-137 and strontium-90.
The best elucidation and advocacy of the Thorium idea is by the Thorium MSR Foundation (Stichting Thorium MSR) directed by Gijs Zwartsenberg, Dr. Kors Bos, and Dr. Sylvia Barlag. Launched in 2016, and based in the Netherlands.
- Site: https://www.thmsr.com/en/lftr/
- Twitter: https://twitter.com/thmsr_nl
- Contact: g.zwartsenberg [at] gmail [dot] [com]
They make a brilliant presentation for the technology, by explaining in a simple way and offering a coherent set of arguments. The main one being that it is impossible for a Liquid Fluoride Thorium Reactor (LFTR) to melt down or explode.
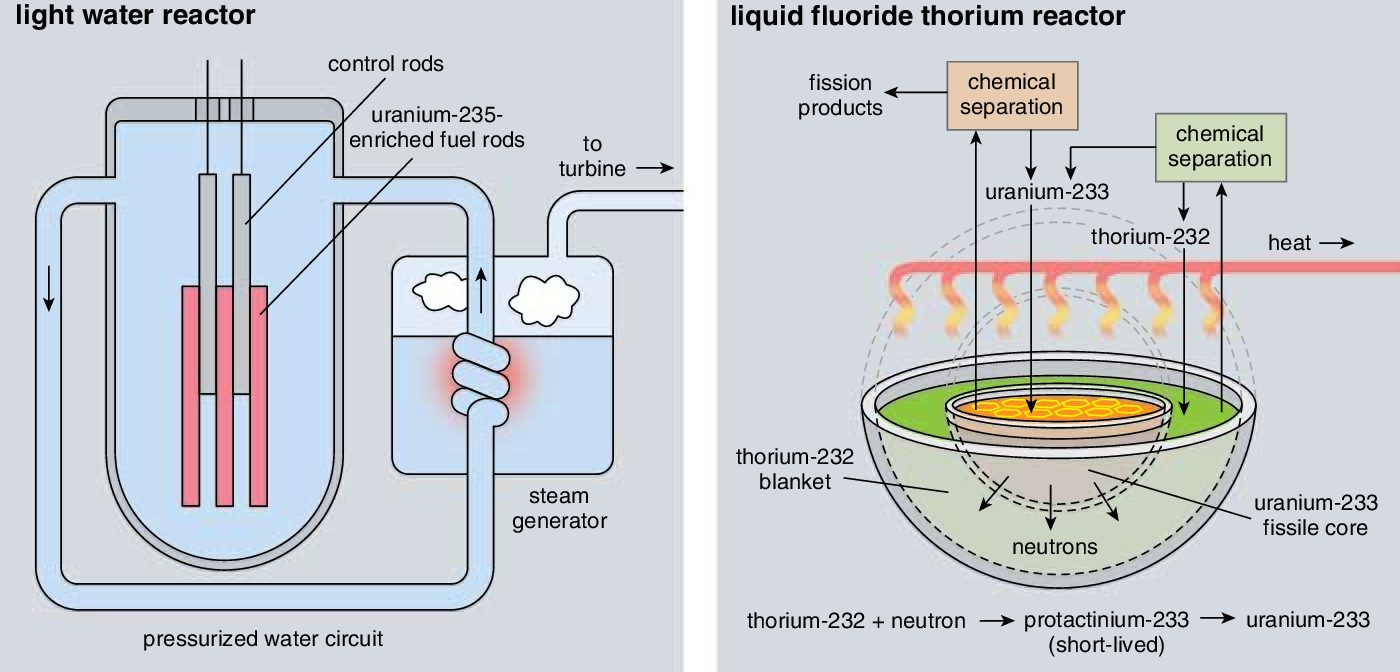
Usually the name LFTR is used for a design that includes two separate molten salt cycles that are combined in a single reactor. The first is the core fluid, the second is called the ‘blanket’. Both contain a different mixture of molten salts with either uranium (core) or thorium (blanket) as its key component. This design is also called a ‘two fluid LFTR’.
In this two fluid LFTR , the uranium in the core serves to generate heat for electricity generation, but also delivers the neutrons that turn the thorium in the blanket into new uranium. This happens when the uranium in the core fissions and releases neutrons that are absorbed by the thorium nuclei in the blanket. The thorium then decays (=transforms) into new fissile uranium. This new uranium is chemically separated and transported to the core, and the process starts all over again.
Because the fuel is liquid, it is possible to keep an optimized mixture at all times. The liquid state makes it easy to separate out useful, as well as unwanted by-products. This is an important advantage over solid fuel, which is difficult to manipulate once inside the reactor. Solid fuel ‘traps’ unwanted fission by-products inside its structure.
The salt mixture considered to be optimal for use in the LFTR consists of lithium fluoride and beryllium fluoride (LiF-BeF), and is commonly referred to as ‘FLiBe’. It has a high boiling point of 1430°C which enables it to remain liquid at high temperatures without turning to steam. It also serves as a neutron moderator. A neutron moderator’s function is to slow down fast neutrons, because neutrons that are too fast are not useful for sustaining the nuclear chain reaction. This does mean however that the LFTR needs an installation to clean and maintain the FLiBe mixture, which runs parallel to the reactor component (Ingersoll, et al., sd, p. 5) , (Carpenter, 2003, p. 7) , (LeBlanc, 2009, pp. 1644,1645), (Sohal, et al., 2010, p. 8) , (Hargraves & Moir, 2010, pp. 307,308), (Hart, 2011, p. 17) , (Sorensen, 2012) , (Kloosterman, 2012).
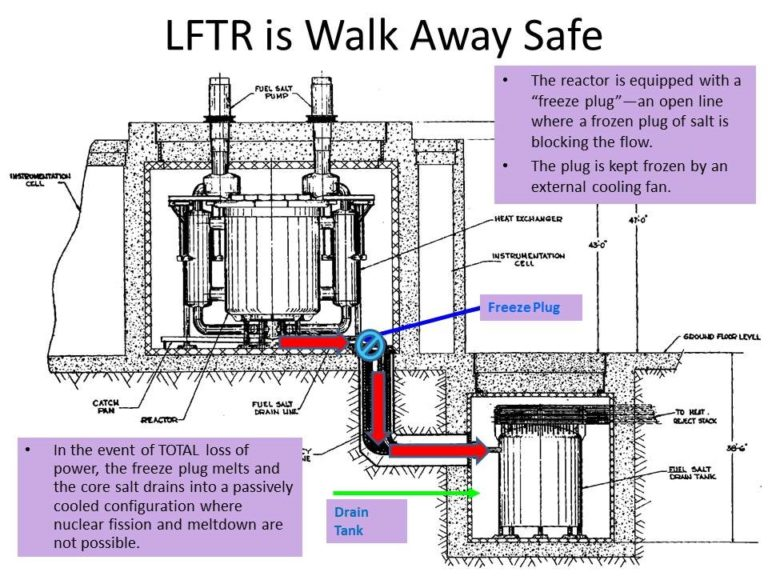
Their arguments are succinct.
Abundance: a year of electricity for a medium sized city (1 gigawatt year, roughly the amount of electricity for a city of one million inhabitants, living by western standards) can be produced with one tonne (1000 kg) of natural thorium. More: https://www.thmsr.com/in-depth/abundant/
Safety: Contrary to most of today’s reactors, the molten salt reactor is not pressurised and contains no water: there is nothing that could cause an explosion. Molten salt reactors therefore also have no ‘driving mechanism’ that would be able to spread the ionically bound radioactive components. More: https://www.thmsr.com/in-depth/safe/
Cleanliness: the waste stream of MSR’s consists of ‘waste only’ – it contains virtually no actinides, comes in very small amounts and will be relatively easy and affordable to dispose of responsibly. More: https://www.thmsr.com/clean/
Proliferation Resistance: The countries with the ability to develop MSR-programs are countries that presently already master the uranium fuel cycle. Countries that have developed those skills and infrastructures already have the possibility to produce weapon material, and developing MSR technology does not add proliferation risk. More: https://www.thmsr.com/in-depth/proliferation/
Interoperation: the MSR can in principle automatically load follow due to some specific properties it has by nature. This will allow for flexible cooperation with intermittent energy sources like sun and wind (this same property has also been described as a safety feature). This means that molten salt reactors could provide a long sought solution to what many consider to be the Achilles heel for renewable power sources: the sun doesn’t shine at night, the wind doesn’t always blow.
Heat Replacement: The primary energy delivered by MSR’s is heat that the molten salt delivers at 550-700 degrees Celcius. This high temperature allows for higher efficiency in electricity generation by employing so called “Brayton cycle” turbines. More: https://www.thmsr.com/in-depth/heat/
Cheapness: Factors contributing to a lower cost profile are simplified construction including less expensive safety systems, modular construction, simplified fuel and waste handling, higher energy efficiency, and the potential for additional processes and earnings made possible by the thorium MSR’s high operating temperature, among others. More: https://www.thmsr.com/in-depth/costs/
Could We Combine Thorium MSR With A Small Modular Design?
Thorium molten salt reactors (TMSRs) and Rolls Royce small modular reactors (RR SMRs) are two different types of advanced nuclear reactors with their unique characteristics. Combining the features of TMSRs and RR SMRs could potentially yield a hybrid reactor design with some of the benefits from both technologies. Let's first briefly explain each type of reactor.
Let's look at the overview again, for clarity:
- Thorium molten salt reactors (TMSRs): These use thorium as the primary fuel and a liquid mixture of salts as both the fuel and the coolant. Thorium is abundant and less proliferation-prone compared to uranium and plutonium. TMSRs operate at high temperatures, are passively safe, and can potentially produce less long-lived nuclear waste.
- Rolls Royce small modular reactors (RR SMRs): These are designed to be compact and modular, with a capacity of around 440 MWe per module. The RR SMR design is based on pressurized water reactor (PWR) technology, using enriched uranium as fuel and water as the coolant. The small size and modular design make it easier to construct, transport, and deploy, allowing for more flexible and cost-effective nuclear power generation.
Combining TMSR and RR SMR technologies could involve incorporating the advantages of molten salt fuel and coolant, along with thorium fuel, into a compact, modular reactor design similar to the RR SMR. This hybrid design could have the following features:
- Compact size and modularity: Retain the small size and modular approach of the RR SMRs, enabling easier construction, transportation, and deployment.
- Thorium fuel: Use thorium instead of enriched uranium as the primary fuel, taking advantage of its abundance, reduced proliferation risks, and potential for reduced long-lived nuclear waste.
- Molten salt coolant: Replace water coolant with a molten salt mixture, allowing for higher operating temperatures, improved thermal efficiency, and passive safety features due to the nature of the coolant (such as negative temperature coefficient of reactivity and passive decay heat removal).
- Simplified design: The use of molten salt coolant and fuel may simplify the overall design, reducing the need for certain components like pressurizers and steam generators, thereby reducing the complexity of the system.
- Fuel cycle flexibility: The hybrid design could potentially utilize a liquid-fueled or solid-fueled core, depending on the desired fuel cycle and proliferation resistance requirements.
A small modular thorium molten salt reactor (SM-TMSR) would combine the advantages of thorium fuel, molten salt technology, and modular design to create an innovative and efficient nuclear power generation system. Here is a theoretical structure and key features for such a reactor:
- Compact core: The SM-TMSR would have a compact reactor core where thorium fuel is dissolved in a molten salt mixture (e.g., lithium fluoride and thorium fluoride) that also serves as the coolant. The core size would be small to maintain the modular concept.
- Fuel flexibility: The reactor could be designed for various fuel cycles, such as thorium-uranium or thorium-plutonium, depending on the desired breeding ratio and proliferation resistance requirements. Additionally, it could be designed for liquid or solid fuel, where solid fuel would be in the form of coated fuel particles suspended in the salt coolant.
- Passive safety features: The SM-TMSR would have passive safety features, including a negative temperature coefficient of reactivity, passive decay heat removal, and a freeze plug system, ensuring the reactor would safely shut down and cool itself without active intervention.
- High-temperature operation: The molten salt coolant would allow for high-temperature operation, typically between 600-800°C. High operating temperatures would improve thermal efficiency and make the SM-TMSR well-suited for various applications, such as hydrogen production or process heat.
- Heat exchanger and power conversion system: The hot molten salt coolant would flow through a heat exchanger, transferring its heat to a secondary salt loop or another working fluid. This secondary loop would then transfer heat to a power conversion system, such as a steam turbine or a closed-cycle gas turbine, to generate electricity.
- Modular design: The SM-TMSR would be designed in a modular fashion, with multiple small reactor units that can be easily manufactured, transported, and assembled on-site. This approach would reduce construction times, lower costs, and allow for flexible power generation capacity.
- Fuel handling and reprocessing: The SM-TMSR could be designed with online fuel processing capabilities, allowing for continuous removal of fission products and the addition of fresh fuel without shutting down the reactor. This feature would help maintain fuel efficiency and reduce the amount of nuclear waste generated.
- Materials and corrosion resistance: The SM-TMSR would require the use of materials that are compatible with and resistant to corrosion by the molten salt coolant, such as Hastelloy-N or other advanced materials.
- Waste management: Due to the thorium fuel cycle and efficient fuel utilization, the SM-TMSR would generate less long-lived nuclear waste compared to traditional uranium-based reactors.
- Proliferation resistance: The use of thorium as the primary fuel reduces the proliferation risks associated with the reactor, as thorium-232 does not readily produce weapons-grade material.
Assuming that the SM-TMSR is designed to be compact and modular, the output capacity of a single module could range from 50 to 300 MWe. The total capacity can be scaled up by deploying multiple modules at a single site, depending on the energy demand.
The waste produced by an SM-TMSR would be lower in volume and have a shorter half-life compared to traditional uranium-based reactors. This is due to the thorium fuel cycle's higher fuel utilization and burn-up rates. However, the exact amount of waste produced would depend on the fuel cycle, fuel processing techniques, and reactor operation parameters.
The modular design of an SM-TMSR should enable faster construction compared to traditional large-scale reactors. If we use the construction time of current small modular reactors (SMRs) as a reference, the construction time for an SM-TMSR could range from 2 to 5 years, depending on the site preparation, manufacturing, transportation, and assembly processes.
The cost of an SM-TMSR would depend on various factors such as research and development, licensing, material selection, manufacturing, and construction. It is challenging to provide an accurate cost estimate without a detailed design and cost analysis. However, small modular reactors are generally expected to have lower upfront capital costs compared to large-scale reactors, due to their smaller size, modular construction, and potential for mass production. The cost of electricity produced by an SM-TMSR would likely be competitive with other advanced nuclear technologies, but the exact cost per MWh would depend on the specific reactor design and operational parameters.
The International Race Is On, and England Isn't
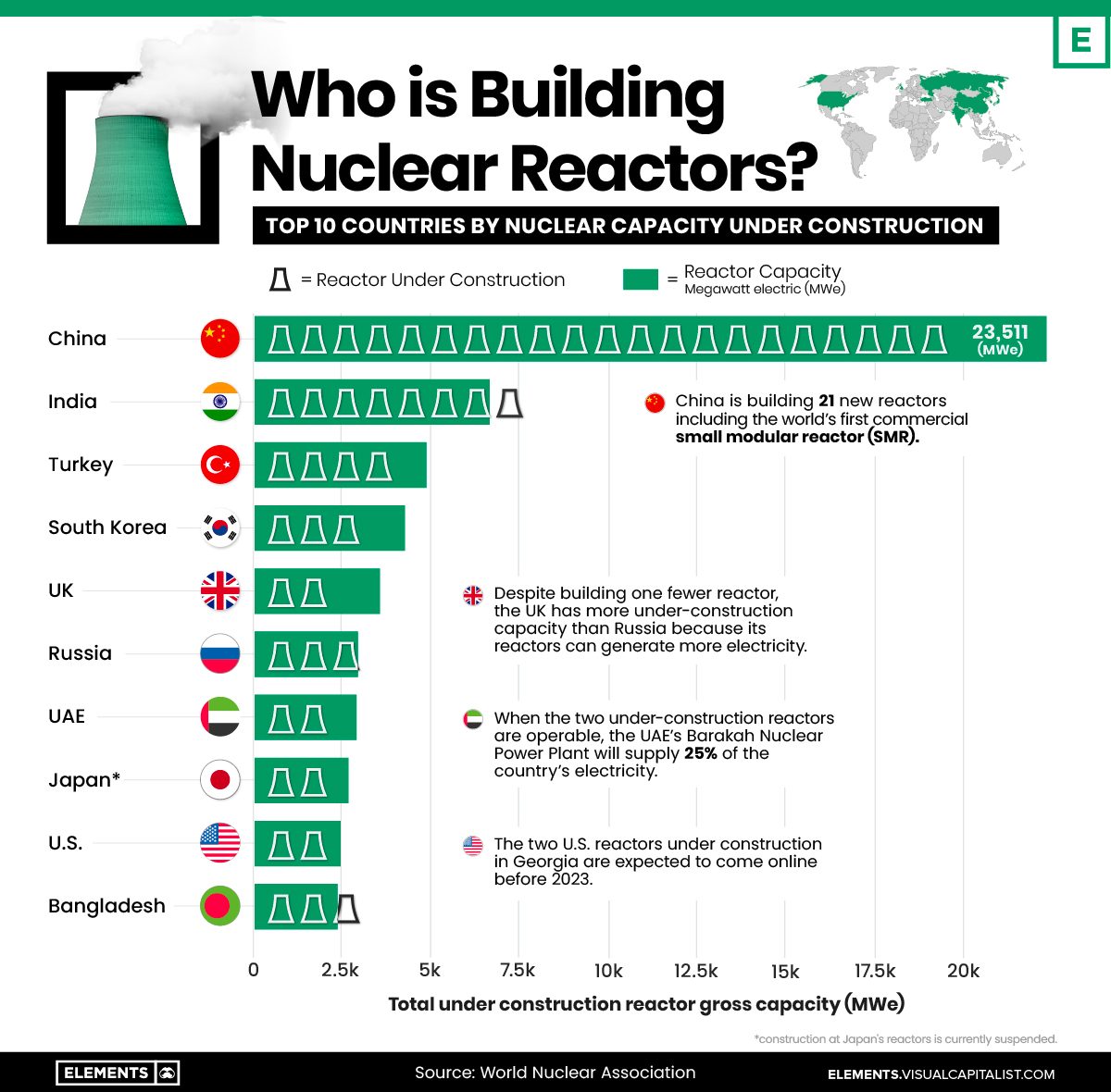
Whatever you may think of them, the two biggest boosts to Thorium power have come from Bill Gates and Andrew Yang.
Gates and Warren Buffet's company TerraPower (https://www.terrapower.com/) are working on a production platform named Natrium (https://natriumpower.com/).
Yang demanded the US have a reactor by 2027: https://thebulletin.org/2019/12/fact-check-five-claims-about-thorium-made-by-andrew-yang/ .
MSR design, has been, or is now being done, in the United States, United Kingdom, Germany, Brazil, India, Indonesia, China, France, the Czech Republic, Japan, Russia, Canada, Israel, Denmark and the Netherlands.
To date, the largest ongoing investment in Thorium has been in India, which makes entire sense on the basis the country owns the largest natural reserves of fuel. 62 reactors have been planned since 2012. It has had a fast breeder reactor at Kalpakkam since 2002.
Thorium Power Canada proposed a 10 MW demonstration reactor in Chile could be used to power a 20 million litre/day desalination plant.
China now leads everyone, and has the Gobi molten salt reactor at Wuwei operated by the Shanghai Institute of Applied Physics (SINAP): https://www.nature.com/articles/d41586-021-02459-w
The question, as always, is, why is the country who first gave the world commercial nuclear power not ready to give it better nuclear power? Why is the entire EU and Anglosphere so focused on hippy pipedreams for this insane apocalyptic quasi-religion of "net-zero" and climate change?
Moving from hydrocarbons to more sophisticated means of generating energy is no bad thing and a smart idea, regardless of why; there is no need for further discussion on the grounds. One side might resemble a Millenarian death cult, and the other might just think it's time for an upgrade. Energy underpins everything else. Having an abundance of it is good.
How do you provide for it? If ones has any aptitude for simple arithmetic, five to ten minutes with the figures makes it self-evidently clear there is absolutely no possibly way any of the "ideas" being pursued by our political leaders have any hope of "solving" the crises they claim are close to catastrophically destroying a 4.6B year-old planet. Even if they did, life would be unlivable on it afterward from the measures taken to prevent them.
60% of all the world's power needs need to be supplanted. 16,000 TWh across the globe (16,093,920 GWhh).
70% of renewable power comes from hydroelectric. The biggest thing we have - the Three Gorges Dam - produces 112 TWh.
There is absolutely nothing, at all, anywhere, in this absurd bag of green tricks which can make the slightest dent in the demand.
Which leaves the nuclear route.
Which has two tracks. What we do now in the 4th and 5th generation, or the other track we didn't pursue because it wasn't good for weapons. The world's two most populous countries have switched on to it out of sheer need. The curiosity is how quickly the West well.
All that is left afterward is the configuration: lots of big stations, or many smaller per-city stations?